Issue:January/February 2018
GENE-EDITING TECHNOLOGY - How CRISPR-Cas9 Technology Will Play a Vital Role in the Future of Human Therapeutics & Drug Discovery
INTRODUCTION
The burgeoning gene editing market is already projected to reach $7.5 billion by 2024. Contributing to this growth are the intensified research and development (R&D) efforts by pharmaceutical and biotechnology companies, primarily due to the role that gene-editing technologies can play in the emerging area of personalized medicine. Indeed, a recent review suggested that known mutations in over 3,000 genes in the human genome can be linked to genetic disorders or a disease phenotype. Recently, the CRISPR-Cas9 technology (Clustered Regularly Interspaced Short Palindromic Repeats – and associated nuclease) has emerged as not only a potential therapeutic itself but also a powerful new research tool. Although initially identified in the mid-1990s to early 2000s as a form of bacterial immunity capable of editing genes, it wasn’t until 2012 that several groups – notably the laboratories of Jennifer Doudna, Feng Zhang, and George Church – demonstrated that a version of this process could be utilized to edit any gene in any cell, including human cells. Since then, CRISPR-Cas9 gene editing has emerged as an essential tool within the research community, and it is changing the way scientists are able to conduct experiments. Let’s explore how this technology can be utilized in research efforts toward the development of new therapies and how it will play a vital role in the future of biopharma and drug discovery.
THE CRISPR TOOLBOX
Although initial CRISPR experiments documented its use for performing simple DNA edits, applications now include the ability to delete genes, transiently inhibit (CRISPRi) or activate (CRISPRa) genes, insert entire genes, and edit regulatory regions with relative ease in a wide range of cell types. Furthermore, CRISPR can be utilized to screen entire gene families of genetic pathways, or to study gene regulation through the identification and analysis of promoter and enhancer regions in non-coding regions of the genome. The adaptability of CRISPR is leading to its widespread adoption within the research community. Following this trend, a recent industry survey conducted by Synthego, a genome engineering solutions company, showed that 87% of new CRISPR users are also new to gene editing as a whole, indicating the tremendous interest in the process’ simplicity and prevalence, as compared to other techniques.
GENE EDITING BEFORE CRISPR
Despite the high adoption rate of CRISPR gene editing for use in human therapeutics and drug discovery, there is still a percentage of scientists utilizing previously developed technologies for this purpose. For example, Meganucleases, Zinc Finger Nucleases (ZFNs), and transcription activator-like effector nucleases (TALENs) are all technologies developed for gene editing as alternatives to viral delivery systems for therapeutic in vivo editing of human cells. However, each of these alternatives to CRISPR depends on a more complex system for targeting and editing genes and are typically less efficient. In addition, they are not as malleable as CRISPR and so cannot be easily repurposed for other applications. Similarly, RNA silencing technology – also known as RNA interference – has long been used for drug discovery research to knockdown genes in cell lines in order to study drug targets or generate models for disease. However, this approach is far less efficient than using CRISPR: gene silencing using CRISPRi is more pervasive due to its less transient nature, and permanent gene knockouts are relatively simple to generate and can be stored for later use. CRISPR is not the only solution for conducting effective gene editing research; however, it does provide distinct advantages in terms of time, ease of use, and application flexibility that are important for scientists to consider for their research projects.
THE ROLE OF CRISPR IN HUMAN THERAPEUTICS
As an alternative to viral delivery systems and TALENs, CRISPR-Cas technology represents a potential gene therapy delivery system in its own right. Currently, many groups are focused on utilizing CRISPR to perform either in vivo editing of human cells – everything from the eye, to neurons and liver cells – or for performing ex vivo therapies. CRISPR could also potentially be used to undertake germline editing of cells and embryos for therapeutic use.
CRISPR-Cas9 genome editing in vivo refers to the delivery of CRISPR components directly to diseased cells in a living organism, such as a human being. Until recently, only viral vectors or TALENs could be utilized for this approach. Therapies relying on an in vivo editing approach have the advantage that they may be able to target and alter multiple cell types (or organs) at once. A recent report showed that delivery of CRISPR-Cas9 components directly into mouse liver cells in a model of human hereditary tyrosinemia disease could correct disease phenotypes – demonstrating that CRISPR-Cas9–mediated genome editing is feasible in adult animals and has the potential for correction of human genetic diseases. Due to the limited number of CRISPR-Cas9 delivery systems available, an early focus of in vivo editing, for example, has been to treat inheritable diseases of the eye by injecting CRISPR-Cas9 components directly into the eye. Recently, CRISPR medicine company Editas Medicine suggested their first foray into human CRISPR trials would take place this year, but in May 2017, they announced their timeline had been delayed. The proposed study, which will target a rare genetic eye disorder, is now expected by mid-2018.
Ex vivo therapies differ from in vivo therapies in that they involve the removal of a donor cell population, typically from the original host. These donor cells are then genome edited (eg, using CRISPRCas9) in a lab and then transplanted back into the patient. If the edited cells are to be transplanted into a different donor, the cells must be allogenic. This method of CRISPR-Cas9 cell therapy has an advantage over in vivo approaches as the CRISPR-Cas9 components can be delivered to the target cell population using a variety of approaches, including electroporation, lipid transfection, or with viral vectors. Because of this, very high editing rates can be achieved. In addition, the dosage to the patient can be controlled more easily than it can be with in vivo-based therapies.
In recent months, the first instance of human embryo editing occurred as well as the discovery of a modified version of CRISPR, which can be used to track RNA in live cells using RNA-targeting Cas9. This methodology could potentially allow doctors to repair molecular mistakes resulting in diseases such as myotonic dystrophy types 1 and 2, the most common form of hereditary amyotrophic lateral sclerosis (ALS) and Huntington’s disease. These discoveries demonstrate the breadth of research currently being performed using CRISPR.
As CRISPR continues to make headlines as a potential cure-all for diseases from Huntington’s disease to cancer, it is important to differentiate between what is possible with the gene-editing tool at present, and its future potential.
Current roadblocks surrounding CRISPR’s immediate advancements in disease elimination include repeatability in experiments and approval in human clinical trials, which are still years away from becoming routine in the United States. The first human trial involving CRISPR in the US was reviewed and given approval by the US National Institutes of Health (NIH) Recombinant DNA Advisory Committee (RAC) in June 2016. However, this is not the final hurdle; the proposal from the University of Pennsylvania will still need to be approved by the US FDA before the study can move forward. Once full approvals are in place, the study will take an estimated 2 years to complete. Three trials involving CRISPR are currently being planned by researchers at Peking University in China; these will investigate CRISPR’s efficacy against bladder, prostate, and renal-cell cancers. Each of these studies across both China and the US are in the very early stages, and most do not yet have approval, meaning that there is a long way to go before therapy involving CRISPR is commercialized and used within the clinic.
Despite these hurdles, companies focused on a CRISPR medicine approach, such as CRISPR Therapeutics, Intellia Therapeutics and Editas Medicine continue to develop the technology with a clear focus on the dynamic combination of pharmaceuticals and gene editing, propelling future advancements in the field. A new player, Casebia Therapeutics, was derived from a 2015 partnership between Bayer and CRISPR Therapeutics, combining their expertise to make headway in drug discovery. To form this initiative, proprietary gene-editing technology from CRISPR Therapeutics was combined with Bayer’s knowledge rooted in protein engineering and disease-specific matters. This strategic partnership pioneered the move toward the development of targeted delivery systems and novel treatment options for diseases. Editas Medicine is another company combining pharmaceutical and gene-editing expertise to develop specific therapies based on CRISPR technology. In 2015, Editas entered a strategic collaboration with Juno Therapeutics, a biopharmaceutical company, to combine its CRISPR technology with Juno’s experience in creating chimeric antigen receptor and high-affinity T-cell receptor therapeutics to develop cancer management and treatment options.
THE ROLE OF CRISPR IN DRUG DISCOVERY
Currently, CRISPR is still largely confined to the basic research stage of the drug discovery process. However, given its ease of use, CRISPR’s potential spans each stage of the drug discovery process; from the identification and validation of new therapeutic targets, to investigations surrounding mechanisms of action, and the creation of screens to identify genes that regulate cell survival processes. Although there remains a great deal for the pharmaceutical industry to learn about contemporary gene editing, there are several applications that standout as areas for growth over the coming years.
Antiretroviral Therapy
Retroviruses are a group of single-stranded positive-sense RNA viruses with a DNA intermediate; retroviruses insert a DNA copy of their RNA genome into the host cell in order to replicate. Examples of retroviruses include HIV and human T-cell leukemia virus (HTLV). Research into retroviruses has made significant progress using CRISPR. For instance, in a 2016 study, a team of researchers were able to locate and remove the HIV genome from infected T cells with no adverse effects to the cells, which continued to grow and divide as normal. In addition, following the removal, the T-cells appeared to remain immune to new HIV infections in the future. Most viruses create proteins by transcribing DNA into RNA, while retroviruses utilize a different method. RNA is reverse-transcribed into DNA and is then integrated as a provirus into the genome of the host cell. Then, the provirus moves through the typical transcription and translational process, expressing the virus’ genes. These retroviruses have many modern-day applications, such as cancer research and gene therapy.
Disease Model Organisms
The key to successful drug development is the availability of suitable model systems with which to make early drug development decisions. Traditional disease models include in vivo applications using rodents, and in vitro experiments using human cell lines. CRISPR has enabled mouse genomic core facilities around the world to exponentially speed up their development of transgenic mouse development. In some cases, transgenic mouse development for gene knockouts, knockins, or single nucleotide variants (SNVs) that once took 6 to 12 months can now be completed in less than half the time.
CRISPR can also advance the use of novel animal models for drug development research. Creating a new disease model can be a laborious process demanding considerable financial investment – and even then, the task is often limited to a few species that come equipped with a good tool kit for genetic manipulation. One such novel model organism is the ferret. CRISPR has enabled researchers to engineer the genome of ferrets in order to modify their susceptibility to flu infections, a critical change since unlike rodent models, ferrets sneeze when infected – a much more human-like response. Moreover, CRISPR also allows traditional model organisms, such as Zebrafish and C.elegans, to be engineered in a much shorter timescale than previously possible. The use of these organisms as models for understanding human cell biology, development, and neurobiology may negate the need for more time-intensive and costly mammal models in the future.
Another benefit to utilizing CRISPR to develop disease models is its ability to be used for multiplex gene editing. For example, CRISPR can be utilized to make multiple genetic changes at once, which more closely approximates human pathology. Many diseases are multigenic as opposed to monogenic, and these new models can contribute substantially to ensuring drug evaluation is more accurate and translational.
Cell Line Development
In addition to the generation of whole animal models for disease research and drug discovery, the generation of knockout or knock-in cell lines can also be a critical tool in the process. For example, the generation of cancer cell lines for modeling different cancer genotypes are useful for screening potential and existing drugs for sensitivity. Alternatively, individual gene deletions, SNVs, or combinations of these can be generated using CRISPR in established cell lines in order to generate disease genotypes or phenotypes for use in drug discovery. Furthermore, CRISPR-Cas9 gene editing can be applied to embryonic stem cells in order to produce disease genotypes and subsequent phenotypes when programmed to develop into desired cell types or organoids. Through this approach, functional organ tissues can be modelled in vitro and tested for sensitivity and reactivity to drug candidates.
FUTURE CRISPR-BASED THERAPIES & CONSIDERATIONS
Taking into consideration the cases and applications of CRISPR we have already discussed, it is clear that future progress and impact with regard to drug discovery will center on chimeric antigen receptor (CAR) T-cells, or receptors that have been engineered onto a T-cell, and stem cell research. With a technique known as adoptive cell transfer, CAR cells are currently being tested as a potential cancer therapy. Researchers remove and modify T-cells, manipulating them to express receptors specific to the form of cancer a patient possesses. From there, the T-cells are reintroduced back into the patient, where they can “seek” and destroy cancer cells.
Research surrounding cancer therapy using T-cells continues to become more prevalent. In July 2017, the Independent Citizens Oversight Committee (ICOC) of the California Institute for Regenerative Medicine approved a $5.8-million award to researchers at the University of California San Diego School of Medicine to develop a new immunotherapy in which patients’ cells would be equipped with a special receptor to recognize and target cancer stem cells, whose survival abilities often render standard therapies ineffective or short-term.
CRISPR has made substantial strides in furthering research and drug discovery within the past few years, and the role of pharmaceutical companies throughout the process will continue to grow as these breakthroughs are made. One important thing for researchers in the areas of pharmaceuticals and drug discovery to remember is that CRISPR is not a one-size-fits-all technology. It is important for scientists to examine each desired goal and projected outcome to fully understand the most effective methods for conducting gene editing. We have a lot to learn as we step into the intersection where CRISPR and pharma converge, but the technology’s pervasive and efficient nature has us on the right track for a successful future in the world of drug discovery.
To view this issue and all back issues online, please visit www.drug-dev.com.
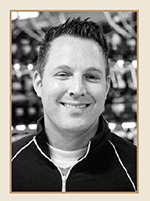
Dr. Kevin Holden is the Head of Synthetic Biology at Synthego. He is a PhD-level scientist trained in molecular and microbiology with more than 10 years of industrial biotechnology experience. He is skilled at utilizing synthetic biology approaches, genome engineering, enzyme evolution, and metabolic engineering, and has spoken frequently about CRISPR at numerous industry events.
Total Page Views: 4880