Issue:March 2017
AAV VECTOR MANUFACTURING - Challenges & Opportunities in the Manufacturing of AAV Vectors Used in the Delivery of Gene Therapy Treatments
INTRODUCTION
Significant advances in the specific targeting of delivery vectors and the increased therapeutic efficacy of such vectors for gene delivery have been made, stimulating major interest in the development and commercialization of therapeutic products focused on gene therapy indications. In the past few years, there have been a large number of positive clinical outputs for gene therapy-based products spanning broad therapeutic areas, including CAR T-cell immunotherapy, oncology, and regenerative medicine based on monogenetic diseases. In terms of gene delivery, viral vectors have emerged as the preferred vehicles of choice, used in 48% of the 483 current on-going gene therapy trials.1
Of the gene therapy products in development, recombinant Adeno-Associated Virus (AAV)-based vectors are currently the most widely used and show the greatest potential for delivery in gene therapy indications.1-3 The first rAAV-vector-based clinical trial was performed 20 years ago; a Phase I study delivering a CTFR transgene via an Raav vector to adult cystic fibrosis patients with mid lung disease.4 In 2015, there were 103 rAAV vector-based products reported to be in development, a number that is expected to increase further in the next few years.1 The preferred use of rAAV vector systems is due, in part, to the lack of disease associated with the wild-type virus, the ability of AAV to transduce non-dividing as well as dividing cells, and the resulting long-term robust transgene expression observed in several Phase I/II trials.5 Furthermore, different rAAV vector serotypes, either naturally occurring or hybrid/synthetically derived, can be exploited to specifically target different tissues, organs, and cells, and help evade any pre-existing immunity to the vector, thus expanding the therapeutic application and commercial potential of AAV-based gene therapies.6
Whilst the majority (74%) of AAV-based therapeutic products are in early to mid-phase clinical development, a number of promising clinical outputs have helped progress the pipeline of possible AAV-based products. In 2012, the Dutch-based company UniQure was granted marketing authorization in Europe for Glybera®, an AAV1-based gene therapy for the treatment of adult patients diagnosed with familial lipoprotein lipase deficiency (LPLD). The recent clinical successes of AAV-, and other viral vector-based gene therapies has fueled significant investments into the sector; as such, there is growing demand for clinical-grade good manufacturing practice (GMP) production solutions for these viral vector products.
THE NATURE OF THE CHALLENGE
The development of manufacturing processes for novel biotherapeutics is time consuming and expensive. Recombinant viral vector production is seen as complex, with the production scale-up regarded as a major challenge technically, and a large barrier for commercialization.
Reported clinical doses for AAV-based viral vectors range from 1011 to 1014 genomic particles (vector genomes; vg) per patient dependent on therapeutic area.1,3,6 From a wider gene therapy development perspective, current scale-out approaches fall short of supplying the required number of doses to allow later Phase (II/III) trails to progress, thus retarding the development of gene therapy products. This is supported by the fact that the majority of clinical studies have been very small, performed on <100 patients (and in some cases <10), using adherent cell transfection processes that generate very modest amounts of product. When predicted amounts of virus required for later phase development are compared to current productivities (ca. 5x 1011 vg from single 10 layer cell factory), there is real concern that this approach will fall short of the material requirements for late phase and in-market needs for even ultra-orphan diseases, which have high dose and small patient cohorts, let alone more “standard” gene therapy indications.
In the case of AAV, a number of production strategies exist to generate viral vectors; as with all different strategies a number of advantages and disadvantages are associated with each.
STABLE CELL LINES FOR PRODUCTION
Generation of stable engineered cell lines, through the introduction of both regulatory (Rep) and structural capsid (Cap) genes and/or the rAAV genome, give rise to packaging or producer cell lines, respectively. AAV viral vectors are produced from packaging cell lines following transfection of the AAV construct and the co-infection with a helper virus, such as adenovirus (Ad) or Herpes Simplex Virus (HSV) or via a single infection with a recombinant helper viral vector containing the rAAV genome. For producer cell lines, AAV is generated following a single-step infection with an Ad or HSV helper virus. Stable cell lines have been reported to produce relatively high AAV vector genome (vg) particles per cell (up to 10,000 vg per producing cell). Packaging and producer cell lines have been generated using cell lines capable of both adherent and suspension growth, allowing for processes to be developed that utilize traditional tissue culture systems for small scale, combined with larger-scale manufacturing performed in suspension bioreactors. This scalable approach has been used in the production of an AAV1 product for heart failure; here the viral vector has been generated at a 2000-L scale from a HeLaS3 producer cell line, following coinfection with helper viruses.7
Despite the relatively high yield and scalability reported, a number of disadvantages have limited the use of the producer and packaging cell lines in clinical development. The generation of stable lines is technically demanding, extremely time consuming, and needs to be performed for every vector and AAV serotype combination. Clonal characterization and cell line stability is very time consuming and expensive, and furthermore carries potential risk based around cell passage history and the relationship between growth kinetics and vector production. A major concern is the use of helper Ad and hybrid variants in these systems. The production of the helper virus can itself be extremely costly and lengthy, with the need to carefully assess the quality of this critical starting material ahead of use in AAV production. Furthermore, establishing effective removal and clearance procedures to separate the helper virus away from the AAV vector product is not trivial, resulting in complex and costly downstream process development and a potentially high cost of goods.
BACULOVIRUS PRODUCTION SYSTEM
Production of AAV vectors using a Baculovirus (BV) expression system emerged as a consequence of the BV system’s ability to produce complex glycosylated recombinant proteins at high levels in SF9 insect cells at high cell densities. Building on AAV production via a stable cell line system, the BV system was developed to produce viral vector without the need to co-infect with a human helper virus. AAV2 was produced in Sf9 insect cells following co-infection with three recombinant BV vectors, encoding the transgenes for Rep, Cap, and rAAV genome, respectively. Since then, the BV system has been modified and improved, with systems now developed that use a two-vector approach, reducing the complexity of the process. Scalable solutions have also been employed; one approach used cryopreserved BV-infected insect cells that separately carry the required AAV components (rAAV genome, Rep, and Cap genes). The infected cell were used to inoculate a 200-L scale bioreactor containing unmodified insect cells, and released infectious rBV (rAAV, rCAP, rRep) in a continuous manner that subsequently infected the uninfected cells, thus driving a sustained production phase.8
Currently, the AAV1-based drug Glybera is produced using the BV system; however, several drawbacks have limited the use of the BV system to produce AAV vectors in the clinical setting. Overcoming the molecular cell biology aspects needed to produce a mammalian viral vector in an insect cell system using insect virus has major challenges; instability of AAV genes within BV has been reported, along with the inability to assemble AAV particles with the correct stoichiometry of capsid proteins, affecting the infectivity of the produced AAV viral vector. Similar to the stable cell line production systems, there are challenges in clearing and removing the starting and propagated BV from the AAV viral vectors during the downstream purification operation. However, several biopharmaceutical companies are currently using variations of the BV platform, and are actively working on improvements to mitigate the limitations of the BV system, moving toward large-scale clinical rAAV manufacture.
HELPER-FREE TRANSIENT TRANSFECTION
Transient transfection of plasmid DNA into mammalian cells for the production of AAV viral vectors is the strategy most commonly used in clinical grade manufacturing of these viral vectors. rAAV vectors are usually produced in human embryonic kidney 293 cells (HEK293), or HEK293 cell variants following introduction (transfection) of typically three DNA plasmids carrying the regulatory (Rep) and structural capsid (Cap) genes, the rAAV transgene, and the specific genes that provide helper Ad function. If all three plasmids are successfully transfected into a cell, the cell will produce an rAAV vector without the need to co-infect with wild type helper virus. The transfection approach is fairly rapid and versatile and has been used to produce different serotypes of rAAV, as only the gene sequence of the Cap genes has to be altered to produce the various serotypes. Furthermore, modification of the transgene plasmid allows for both single- and double-stranded (self-complementary) forms of the vector to be generated.
Again, the main challenge to this approach is the inherent lack of scalability due to the use of adherent HEK293 cells. As such, adherent cell systems require a scale-out approach based on the linear expansion of 2D surface area, rather than the volumetric 3D scale-up approach usually employed in the production of biopharmaceuticals. In order to produce and purify the required vector genome (vg) particle numbers of >1×1016 vg to support mid- to later-stage clinical trials, over 500 Hyperstacks™ (a Hyperstack has 36 layers and total surface area of 18,000 cm2) would be needed. Whilst possible, this approach is not a viable option for most manufacturing facilities due to facility footprint, cost, and man power limitations. Work is underway to develop truly scalable, regulatory compliant, production solutions for rAAV vector generation. HEK293 cells have been adapted to suspension growth in animal component-, serum-free, and antibiotic-free media systems, with optimization of transfection conditions evaluated in shake flask, rocking, and stirred-tank bioreactor systems.
DOWNSTREAM VIRAL VECTOR PURIFICATION
Irrespective of vector production processes and scales, the ultimate goal of for AAV vector manufacturing is to have robust downstream purification that generates final clinical material that has high titre, high potency, and high purity. Due to the emerging nature of the viral vector field, most downstream approaches are based around traditional laboratory processes that are not scalable or suitable for clinical-grade manufacture. Limitations exist throughout the downstream purification of viral vectors; these include: the harvesting of the producer cells, cell lysis procedures to release AAV vector, the clarification and removal of cellular impurities, vector separation and purification, and vector formulation and sterile filtration.
The challenge can be exemplified by the way that genomic-containing vector particles have been traditionally separated from empty particles using gradient ultra-centrifugation. Whilst the resulting material obtained from such a step is of high purity and high potency, the time-consuming nature and complexity of scale up significantly limits the use of ultra-centrifugation within the downstream process. For therapeutic indications, other than those focused at ultra-orphan diseases that require small volumes of material to be purified, the need for processing large volumes requires an alternative method of purification. As such, chromatography-based approaches are being developed using affinity binding and/or ion-exchange steps that provide efficiency, flexibility, and scalability to the purification of AAV vectors. As high-purity, high-potency vectors can be generated using ultracentrifugation approaches, the challenge for a chromatographic purification approach is to generate vectors with the same degree of purity and potency. Regulatory consideration must therefore be given to any process change during the clinical development program. Also, due to the chemical and biological variances observed between the various AAV serotypes, the development of a single downstream platform for all AAV vectors may be unlikely; rather a number of potential solutions are likely to be employed.
ANALYTICAL SYSTEMS FOR THE CHARACTERIZATION OF PRODUCTS & PROCESSES
Developments are underway to improve clinical manufacturing processes and to move into scalable and controllable production and purification systems. However, these are stymied by the lack of processing knowledge of critical parameters at all stages of the manufacturing process, driven by the lack of suitable analytical systems to support development studies, and to interrogate and control production processes, as well as characterize final materials.9 Linked to the manufacturing challenge is the regulatory requirement for the identification, characterization, and batch-to-batch control of the process- and product-related impurities present in highly purified material. Especially challenging are impurities related to the AAV vector products, which closely resemble the vector itself.
AAV vector particles can co-package non-specific plasmid material into the capsid. It has been reported that between 1% and 8% of purified AAV particles contain an incorrect nucleic acid sequence.7 As reported clinical dose ranges vary from 1011 to 1014 genomic particles, there could be as many as 109 co-packaged impurities per dose. These process-related impurities require detailed characterization, along with an evaluation of the potential for the incorrectly packaged DNA to be active in transduced cells.10
Interestingly, most plasmids currently used for transient transfection carry an antibiotic resistance gene to enable plasmid selection and maintenance during their own production. Therefore, removal of antibiotic resistance genes would be desirable for the production of AAV in Helper-free systems. Technology exists, such as Cobra’s patented Operator Repressor Titration (ORT™) plasmid maintenance system, to generate plasmid DNA lacking antibiotic resistance genes.11 These systems eliminate the chance of co-packaging a functional antibiotic-resistance gene into the viral capsid, thus increasing the safety of the product and reducing the burden of characterization of AAV-related impurities.
VISION FOR A KNOWLEDGE-BASED SCALABLE PRODUCTION PLATFORM
There remains a clear need for improved process productivities, and the need to develop manufacturing processes that can be applied to a wide number of AAV-based viral vector therapeutic candidates. Simplistically, the AAV vector is a delivery vehicle for a therapeutic gene, and the manufacturing process is not linked to that gene. Logic therefore dictates that it should be possible to generate platform processes specific for AAV serotypes and even possible to generate processes that can be applied to multiple serotypes. This approach is not unprecedented; the development of platform-based development and manufacturing processes that disconnects production from specific products has had an enormous impact in other biologic areas (ie, monoclonal antibodies), reducing development cost, timelines, and cost of goods, and has also allowed drug developers to significantly expand clinical pipelines in multiple therapeutic areas.
To achieve this for AAV vectors, the underpinning scientific knowledge surrounding critical operational parameters in the manufacturing process needs to be generated and optimized, along with a suite of standard analytical methods. Any platform developed will need to be sufficiently flexible to accommodate multiple AAV vector types. Such development will have long-term benefits to the generation of materials for first-in-man studies, and onward for late-stage clinical studies and ultimately in-market supply.
REFERENCES
1. Gene Therapy Market 2015-2025, Roots Analysis Report (2015).
2. Ginn SL, Alexander IE, Edelstein ML, Abedi MR, Wixon J. Gene therapy clinical trials worldwide to 2012 – an update. J Gene Med. 2013;15:65-77.
3. Okada. Gene Therapy, Tools & Potential Application. 2013;C17.
4. Flotte, et al. Hum Gene Ther. 1996;7:1145-1159.
5. Okada, et al. Methods Enzymol. 2002;346:378-393.
6. Wu, et al. Molecular Therapy. 2006;14:316-327.
7. Hajjar et al. J Card Fail. 2008;14:355-367.
8. Cecchini et al. Hum Gene Ther 2011;22:1021-1030.
9. Wright JF. Biomedicines. 2014;2:80-97.
10. Quality, non-clinical and clinical issues related to the development of rec. AAV vectors; 24 June 2010,EMEA/CHMP/GTWP/587488/2007.
11. Cranenburgh. Et al. Nucleic Acids Res. 2001;29:e26.
To view this issue and all back issues online, please visit www.drug-dev.com.
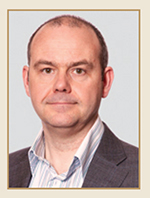
Dr. Daniel C. Smith is Chief Scientific Officer of Cobra Biologics with the responsibility for developing the highest level of scientific excellence across the Group and enhancing Cobra’s DNA, virus, microbial, and mammalian proteins research and development platforms. Prior to joining Cobra, he spent 4 years with the bioProcessUK team at the HealthTech & Medicines Knowledge Transfer Network (KTN), driving the innovation agenda for biologics bioprocessing in the UK as a Knowledge Transfer Manager. Dr. Smith gained his industrial experience at Cobra in a variety of roles progressing from Senior Scientist to Commercial Scientific Development Manager, responsible for developing the strategy for customer’s projects, alongside maintaining scientific oversight of Cobra’s R&D projects. He earned his BSc (Hons) in Biochemistry and his PhD in Molecular Cell Biology. He has more than 20 research publications to his credit; has worked with a number of academic groups across the UK, Europe, and the US; and has extensive academic research experience in cell biology, molecular biology, immunology, and protein biochemistry, with a particular expertise in protein-based toxins and cellular delivery vectors.
Total Page Views: 42652