Issue:June 2017
SPRAY-DRIED DISPERSIONS - Particle Engineering of Spray Dried Dispersions: Considerations for Downstream Processing
SPRAY DRIED AMORPHOUS SOLID DISPERSIONS
Amorphous solid dispersions (ASD) are a common formulation approach for orally delivered small molecule active pharmaceutical ingredients (APIs) whose oral bioavailability (BA) is limited by dissolution rate and/or solubility in the gastrointestinal tract. Some of the foremost reasons this method has become so prevalent are the large potential for gains in bioavailability, easily assessable and mature manufacturing platforms, and straightforward integration of the resultant dispersions into an oral solid dosage form. Given the amorphous form of the API has significantly higher free energy than its neutral crystal form, it will have a higher aqueous solubility, and therefore, a more rapid dissolution rate. For these reasons, it is fairly straightforward to formulate an ASD that has improved BA compared to its crystalline form. However, it can be challenging to formulate a shelf-stable ASD that maximizes absorption potential and at the same time optimizes attributes amenable for downstream processing into an oral solid dosage form.
A common approach to manufacturing ASDs is spray drying (SDD).1 In this process, the API and excipient(s) are dissolved in a common volatile solvent and atomized into a stream of hot gas. As the solvent evaporates from the droplet, the solutes rapidly solidify, trapping the API in an amorphous state within the excipient matrix. A schematic of droplet to particle formation is presented in Figure 1.
The mechanism described in the schematic assumes the droplet is being dried very rapidly (high Peclet number) such that the solute concentrates at the solvent gas interface until an initial skin forms due to solidification from exceeding the solute solubility. Up to this point, the droplet temperature is at its wet bulb temperature. Once a skin is formed, the temperature increases due to a slower rate of solvent evaporation resulting from a slower diffusion of solvent to the particle surface. This slower rate is primarily dependent on the gas temperature, liquid and gas flow rates, and droplet size. Depending on the viscosity, thickness, and radial solvent concentration of the skinned layer, as well as the droplet temperature after skin formation, the particle may inflate or deflate. Presumably, during slower drying kinetics, this skin is relatively thin and more pliable due to a smaller concentration gradient from the surface of the shell inward. This, combined with a lower solvent vapor pressure inside the particle than pressure outside of the particle, causes the particle to collapse in on itself into a “collapsed hollow spheres” geometry. For markedly faster drying kinetics, the skin forms in the same way. However, the thicker shell, due to the higher solvent concentration gradient combined with a higher vapor pressure in the particle due to a higher particle temperature (higher than the solvent boiling point), causes the particle to inflate and sometimes fracture. If the particle fractures, the pressure inside and outside of the particle equalizes, but unlike the slow drying case, the particle does not buckle in on itself because the pressure inside the particle is never at or below the outside pressure until the particle is rigid. The fracture can heal or persist. The resulting particle morphology is the hollow sphere. For asymptotically fast-drying kinetics in a spray dryer, the vapor pressure in the skinned particle builds up very fast, substantially inflating the particle such that the resulting particle wall is very thin. These particles are susceptible to shattering from collisions with other particles, the spray dryer walls, or in the cyclone, which is used to separate the particles from the gas stream. The gas is run through a scrubber, or direct vented if the spray dryer is set up in a single pass mode. If set up in a recycle mode, as is the case for large-scale spray dryers using nitrogen, a large fraction of the gas is processed through a condenser, mixed with a portion of fresh gas and recycled. A secondary drying step is often needed to remove residual solvent to a safe concentration for human consumption and ensure good physical state stability. It is impractical to dry to such a low-solvent concentration during the spray drying process due to short particle residence times in the spray dryer.
FORMULATION CONSIDERATIONS
The amorphous state for most small molecule drugs is thermodynamically unstable and, unless the glass transition temperature (Tg) is unusually high, also kinetically unstable. However, the amorphous state can be stabilized by dilution of the drug in an excipient matrix. Assuming the drug and matrix material are not miscible in the solid state, the dispersed drug has the added barrier of diffusion and phase separation in order to crystallize. By choosing an excipient matrix material that has a high Tg, diffusion of the drug can be slowed and the rate of phase separation and crystallization substantially reduced. This is because the high Tg polymer limits the mobility of the API to diffuse within the excipient matrix given the markedly high viscosity for amorphous glasses below their Tg. As a result, the most common type of excipient in an ASD formulation is a high Tg polymer. Among the most common are neutral cellulosics (eg, HPMC, HEC), enteric cellulosics (eg, HPMCAS, CAP, HPMCP), methacrylates (eg, various grades of Eudragit), and pyrrolidones (eg, PVP, PVPVA). In cases when the API and excipient are not thermodynamically miscible with one another in the solid state, the spray dried dispersion (SDD) is formulated such that the resulting Tg of the mixture, including absorbed water, is at least 10°C to 20°C greater than typical storage conditions. This ensures the drug product will be shelf-stable with respect to phase changes.
The type of excipient matrix in the SDD is also chosen to aid in rapid dissolution, achieving a high supersaturated drug concentration with respect to neutral crystalline drug and sustaining the high concentration by inhibiting precipitation and crystallization of the drug. Choosing SDD formulations that maximize these in vitro dissolution properties can enhance the oral bioavailability of the drug when dosed in vivo. Another key formulation variable is the choice of drug loading in the SDD composing of the excipients preferred for desirable stability and dissolution properties. In general, unless the therapeutic dose is low, it is desirable to maximize the drug loading in the SDD, to minimize the size and number of dosage forms that need to be dosed to achieve the target pharmacokinetic (PK) profile. However, physical stability and dissolution performance nearly always decrease with increasing drug loading. Thus, early in development, an SDD formulation is chosen that has as high of a drug loading as possible while achieving the best overlay in acceptable physical stability and dissolution performance. It is then required to incorporate this SDD formulation into a solid dosage form that at least retains and perhaps improves stability and performance attributes.
As evident, design and integration of an SDD formulation into an oral solid dosage form is a multivariate optimization focused on 1) PK – determined by the drug properties and release from the dosage form; 2) patient compliance; 3) drug substance and product stability; 4) manufacturability, processing throughput; 5) and cost. While each of these metrics is important, it is beyond the scope of this article to describe the numerous aspects of SDD formulation and optimization related to each. The focus of this article is to demonstrate the tunability of SDD particle properties and the resulting impact on the powder flow and mechanical properties for tablet manufacturability of a given SDD formulation. Optimization of these properties is generally performed after selection of a lead SDD formulation and within a process operating space constrained by in vivo performance as well as physical and chemical stability of the drug in the amorphous state. These particular aspects have been extensively reviewed in the literature.2,3
SDD PARTICLE ENGINEERING
In addition to the selection of the composition of the SDD, particle morphology and physical properties can be significantly “tuned” during the spray drying process by changing atomization properties, spray solution properties, and drying kinetics. This enables the formulator to alter the SDD’s specific surface area through particle size, shape, and density, and to modify the surface properties.4 Modification of these properties significantly impacts downstream processing by changing the powder flow and mechanical properties of the SDD. A comprehensive list of the major formulation and process variables and resultant SDD material properties are presented in Figure 2. Given the complexity and interactions between process and formulation variables, a model-based methodology to select and optimize spray drying conditions has been proposed by Dobry et al.5 This type of method enables a formulator to engineer SDD particle properties without disregarding process and formulation constraints by establishing a process operating space based on fundamental engineering models and state-of-the-art characterization techniques.
Examples of several particle morphologies of a placebo SDD composed of PVP-VA are presented in Figure 3. As evident from the SEM micrographs, an extensive range of particle morphologies and particle sizes can be achieved by simply changing the way the SDDs are spray dried. Particles shown in the micrographs were sprayed from solutions and emulsions, demonstrating a large range in particle architectures. Spray drying from solutions is a much more conventional approach for SDDs in oral solid dosage form development, whereas spray drying from emulsions is more common in industries such as food and cosmetics, where it has been extensively used for microencapsulation.6 However, there is a growing interest in the pharmaceutical industry in leveraging spray dried emulsions to engineer particles for pulmonary delivery.7 In addition to spray drying parameters, use of an API inevitably changes the range and magnitude of particle properties that are achievable. However, the majority of amorphous dispersion formulations are constrained to an API loading around 25% or less due to physical stability and in vivo performance decreasing with increasing API loading. At these lower API loadings, the particle material properties are dominated by the properties of the matrix polymer. This is not the case when the API loading in the SDD is higher.
Typically, the simplest development pathway is to optimize the SDD composition and particle properties independently followed by another independent optimization of the drug product formulation and or process. However, by co-optimizing the SDD composition and particle properties and drug product formulation, it is often possible to achieve higher active loading in the final dosage form, potentially reducing pill burden. In addition, other metrics, such as improved in vivo performance and reduction of manufacturing-related risks, can often be achieved. For example, rather than relying on the SDD intermediate to achieve the full performance objectives, performance-enhancing excipients can sometimes be incorporated external to the SDD (eg, in the drug product). Furthermore, the SDD particle properties can be tuned to minimize the need for fillers and other tableting excipients in the drug product.
SDD PARTICLE ENGINEERING CASE STUDY
To illustrate the concept of co-optimizing the SDD and drug product formulation through SDD particle engineering, four SDDs were spray dried from a drug polymer solution of acetone on a single pass Niro pharmaceutical spray dryer (PSD-1). PVP-VA was used as a model SDD composition. The particle properties were tuned by varying the drying rate, atomization mechanism, and conditions and polymer concentration in the spray solution. A heat and mass transport model was used to help select spray drying conditions within a wide operating space to show the potential maximum variance in particle properties that can be achieved by changing these parameters for the given formulation (drug-polymer system). Particle properties were characterized by particle size, measured by laser diffraction, bulk density, and particle morphology by scanning electron microscopy (SEM), and specific surface area measured by the Brunauer-Emmett-Teller (BET) method. The spray drying conditions and resulting particle properties are presented in Table 1.
SEM micrographs of the SDD particles for samples A, B, and C are presented in Figure 2. By changing the particle size through atomization, and particle morphology and particle density primarily through varying the rate of droplet drying (drying kinetics), it is possible to achieve specific surface areas that span an order of magnitude (0.23 – 2.90 m2g-1). Demonstrating that this range in surface area can be achieved, assuming the homogeneity of the starting material is conserved, it has broad implications. This includes the potential for, 1) enhanced in vivo performance for APIs in the amorphous state that are dissolution rate limited; 2) lowered chemical stability of the material since higher surface area provides higher exposure to oxygen, moisture, and additional excipients; 3) decreased physical stability due to higher crystallization rate on the surface of amorphous glasses below their Tg2; 4) improved mechanical properties given that the strength of a compact is determined by it bonding area and strength; and 5) decreased powder flow properties given high surface area powders tend to be small and low density and therefore often are cohesive and consolidate.
Compactability, tabletability, and compressibility (CTC) profiles8 were measured for each SDD to illustrate the impact of particle properties on the mechanical properties of the SDDs. Compressibility is the relationship between the solid fraction of the ejected tablet and the applied compression stress, whereas tabletability compares the tensile strength of a tablet to the applied compression stress. Compactability relates the tensile strength to solid fraction. A tablet will generally have acceptable integrity to withstand further processing and storage with a minimal impact on release rate for a tensile strength of 1.5-2.0 MPa or greater with a solid fraction less than 0.85. Profiles for samples A, B, and C are presented in Figure 4, and the resulting tensile strengths and solid fractions for the compacts compressed with a pressure of 100 MPa are tabulated in Table 2 for all four samples.
As evident by the wide range in tensile strengths (1.6-6.2 MPa) resulting from a peak compression pressure of 100 MPa, the range of particle properties achieved translated to a large range of powder mechanical properties. Although tensile strength of a compact generally correlates to bonding area9,10 and therefore particle surface area (considering these are made from the same material), this was not the case for this sample set. Sample B (the hollow spheres), which had the smallest particle size and second largest surface area, presumably due to differences in the average particle wall thickness, had the lowest tensile strength. Sample A (collapsed hollow spheres), which had the largest surface area, had the largest tensile strength. Cross sections of the compacts compressed at 80 MPa were imaged by SEM to gain insight into the causes. Representative SEM micrographs are presented in Figure 4. These micrographs revealed that particle morphology likely impacted the mechanical properties in three ways. The first mechanism was related to the total bonding area. While this was not quantified and also depends on particle size distribution, the collapsed spheres morphology seemed to consolidate more efficiently than the hollow spheres, likely leading to a greater number of contact or bonding areas. Secondly, for the shattered particles, there was likely a high degree of anisotropy due to the hollow sphere pieces orienting perpendicular to the direction of compression. This will also likely be the case for SDD particles that fracture significantly upon compression. Finally, the particle wall thickness and the extent the particle collapses in on itself will have an impact on the irreversible deformation, and therefore the bonding area. Thick spherical particles are more difficult to deform as compared to thin-walled collapsed spheres. Although this analysis explains differences in mechanical properties for the same SDD formulation through differences in bonding area primarily due to particle morphology differences, a more in-depth analysis is needed when comparing the mechanical properties of different SDD formulations. This is because changes in material properties, such as elasticity, hardness, and brittleness, in addition to particle morphology, change the way a SDD will behave under an applied stress. Furthermore, while CTC profiles provide an initial insight into how a material will behave during high-speed tableting, there are many other aspects that should be considered when formulating a tablet and selecting a process train, including the impact of strain rate, elasticity, and resulting density gradients as a function of not only the mechanical properties but also the tablet shape.
Powder flow properties were considerably impacted by SDD particle properties as calculated by Carr’s Index from each SDDs bulk and tapped density, as well as by measuring the flow function coefficient (FFC) on a Shultze ring shear tester. These results are presented in Table 2. While not an exhaustive approach to characterize powder flow, based on this work, these two measurements appear to be the most informative for drug product formulation and process optimization. Not surprising, the FFC and Carr’s index improved with decreasing surface area. However, these values were more sensitive to particle size than bulk density. Given the small number of samples, the extent to which particle morphology played a role in the powder flow properties was not clear. Sample D, the large collapsed spheres, exhibited the best powder flow characteristics, and depending on the SDD loading in the tablet, formulation, and process train, it could be a candidate for direct compression.
SUMMARY
In conclusion, SDD particle size, morphology, and density (particle wall thickness) can be considerably tuned by the atomization conditions and rate of droplet drying (drying kinetics) in the spray dryer. These particle attributes have a significant impact on several aspects of SDD and oral drug product development, one of which is downstream processing. Significant reduction of scale-up risk and an increase in active loading in the dosage form – potentially reducing tablet size or pill burden – can be realized by co-optimizing the SDD intermediate and drug product for improved SDD powder flow and mechanical properties.
REFERENCES
1. Friesen DT, Shanker R, Crew M, et al. Hydroxypropyl methylcellulose acetate succinate-based spray-dried dispersions: an overview. Mol Pharm. 2008;5(6):1003-1019.
2. Singh A, Van den Mooter G. Spray drying formulation of amorphous solid dispersions. Adv Drug Del Rev. 2016;100:27-50.
3. Williams HD, Trevaskis NL, Charman S, et al. Strategies to address low drug solubility in discovery and development. Pharmacol Rev. 2013;65(1):315-499.
4. Paudel A, Worku ZA, Meeus J, et al. Manufacturing of solid dispersions of poorly water soluble APIs by spray drying: Formulation and process considerations. Int J Pharmaceut. 2016;453(1)253-284.
5. Dobry DE, Settell DM, Baumann JM, et al. A model-based methodology for spray-drying process development. J Pharmaceut Innov. 2009;4(3):133-142.
6. Gharsallaoui A, Roudaut G, Chambin O, et al. Applications of spray drying in microencapsulation of food ingredients: An overview. Food Res Int. 2007;40(9):1107-1121.
7. Healy AM, Amaro MI, Paluch KJ, et al. Dry powders for oral inhalation free of lactose carrier particles. Adv Drug Del Rev. 2014;75:32-52.
8. Tye C, Sun C, Amidon G. Evaluation of the effects of tableting speed on the relationships between compaction pressure, tablet tensile strength, and tablet solid fraction. J Pharm Sci. 2005;94:465-472.
9. Osei-Yeboah F, Chang S, Sun CC. A critical examination of the phenomenon of bonding area – bonding strength interplay in powder tableting. Pharm Res. 2016;33(1):1126-1132.
10. Jain S. Mechanical properties of powders for compaction and tableting: an overview. Pharm Sci Tech Today. 1999;2(1):20-31.
11. Grosshans H, Griesing M, Hellwig T, et al. A new model for the drying of mannitol-water droplets in hot air above the boiling temperature. Powder Technology. 2016;297:259-265.
12. Vehring R. Pharmaceutical particle engineering via spray drying. Pharmaceut Res. 2008;25(5):999-1022.
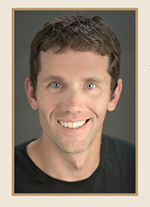
Dr. Aaron Goodwin is a Principal Engineer in the Research and Development department at Capsugel Bend. His responsibilities include platform technology development for enhancement of oral bioavailability for small molecules. Dr. Goodwin has been with Capsugel Bend since 2001. He earned his Bachelor’s degree in Chemistry from the University of Puget Sound and a Master’s degree and Doctorate in Chemical Engineering from Oregon State University.
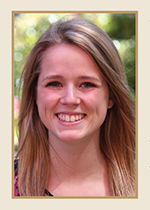
Alyssa Ekdahl is a Research and Development Engineer at Capsugel Bend. Her responsibilities include technology development for oral drug delivery, and her technical focus has been on particle engineering for spray dried dispersions. She has been with Capsugel since 2015 and earned her BS in Chemical Engineering from Oregon State University.
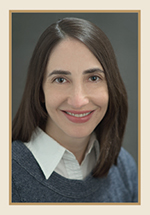
Dr. Deanna Mudie is a Principal Scientist in the Research and Development department at Capsugel Bend. Her focus is on enabling bioavailability-enhancing amorphous spray dried dispersions by designing and developing solid oral dosage form platforms, and investigating novel techniques for evaluating performance of these platforms using in vitro test methods. Dr. Mudie has been with Capsugel since 2016. She earned her PhD in Pharmaceutical Sciences and BSE in Chemical Engineering from the University of Michigan.
Total Page Views: 15642