Issue:October 2013
SPRAY-DRIED DISPERSIONS - Efficient Scale-Up Strategy for Spray-Dried Amorphous Dispersions
ABSTRACT
The increase in low-solubility drugs coming out of discovery labs has fueled the need for new formulation and processing approaches to address bioavailability challenges. Amorphous dispersions, manufactured using spray-drying and hot-melt extrusion, have emerged as a platform technology for mitigating bioavailability challenges. Spray-drying offers particular flexibility in formulation, because it allows use of polymers with high melting temperatures and offers rapid drying kinetics. The availability of small-scale spray-drying equipment and analytical techniques makes it possible to conduct feasibility and process-development work in a bulk sparing manner. In addition, the spray-drying process can be efficiently scaled from milligram to metric ton quantities and has demonstrated commercial viability, making this process broadly applicable in the development and commercialization of amorphous dispersions.
Previous articles contributed by Bend Research have focused on amorphous formulation selection and phase-appropriate formulation and process development approaches.1,2 This paper is focused on scale-up methodology for spray-dried dispersions (SDDs). Two critical focus areas guide scale-up: (1) atomization of the feed solution into droplets and (2) droplet drying. The following describes these focus areas and methodologies for efficient scale-up of the spray-drying process while maintaining the critical-to-quality attributes (CQAs) of the SDD.
INTRODUCTION
To identify a robust operating space during scale-up, an understanding of key spray-drying process parameters and their relationship to the critical-to-quality attributes (CQA) of the SDD is paramount. Specific formulation and process knowledge gained early in the feasibility and development lifecycle can be used to guide development studies and risk assessments that facilitate identification of SDD CQAs. Some common SDD CQAs and the rationale for their criticality are described below.
Particle Size impacts powder handling and tableting and can impact performance, particularly for highly lipophilic compounds where this attribute can be linked to dissolution rate.2
Density or Compressibility impacts powder flow and the ability to manufacture tablets with acceptable performance and mechanical attributes.
Physical State can impact performance and physical stability of the formulation.
Residual Solvent Remaining in SDD can impact the physical stability of the formulation prior to secondary-drying operations.
The attributes of the functional excipients (eg, polymers or surfactants) and other raw materials may result in additional SDD CQAs. For brevity, we will focus on process-related SDD CQAs in this article and not address attributes related to raw materials.
The main goal of scale-up is to ensure that the SDD CQAs are optimized and do not change as larger-scale spray-drying equipment is used and throughput is increased. It is essential to select a robust formulation early in development and to conduct early process-development work with consideration to scale effects. Small-scale experiments, computer simulations, and scale correlations, coupled with risk assessments and process-development experience and knowledge, form the basis of any scale-up strategy and provide a fundamental approach for process-space definition.
A general SDD manufacturing process consists of five steps: (1) solution preparation, in which the drug and excipients are dissolved in appropriate solvents; (2) atomization of the spray solution; (3) primary drying of the atomized droplets in the spray chamber; (4) collection of the SDD particles via cyclone; and (5) secondary drying to reduce the residual solvent to acceptable limits.
If the equipment is well understood and carefully designed and selected, SDD CQAs are typically defined during the atomization and primary droplet drying steps. Figure 1 outlines the key control volume and spray-drying parameters for SDD CQAs. As the figure shows, the liquid spray solution is atomized into a fine spray, consisting of droplets with high surface area, which enables rapid evaporation of the spray solvent. Hot drying gas (typically nitrogen due to the flammability of common spray solvents) contacts the droplets as they are formed, providing the energy source for evaporation and droplet drying.
The scale-up methodology presented here outlines tools and concepts to achieve the target droplet size and drying kinetics from liquid droplets to dried particles across scales. This methodology assumes that the optimal equipment configuration for SDDs (ie, a tall-form spray dryer with gas disperser designed for low turbulence near the nozzle and efficient cyclone collector) is employed across all scales.
ATOMIZATION
Atomization is one of the most critical aspects of the spray-drying process since it controls the droplet size which, combined with the spray solution composition and properties (eg, excipient selection, solution solids loading) defines the final particle size.3 Pressure-swirl and two-fluid nozzles are generally the preferred atomization equipment for SDD manufacture. The polymeric excipients most commonly used may result in spray solutions that are viscous and film-forming, making scale-up challenging with other atomization methods.
To ensure that SDD particle size remains constant during scale-up, droplet size is matched across scales. As spray solution throughput increases with increased scale, the energy input required to maintain the target droplet size also increases. It is critical to understand this relationship early in development so that SDD particle-size targets can be selected that are appropriate for future development and scale-up. Figure 2 illustrates this relationship for a pressure-swirl nozzle atomizing a spray solution containing hydroxypropyl methylcellulose acetate succinate (HPMCAS), a common polymer used to prepare SDDs. Droplet size is shown at increasing solution flow rates for three GEA Niro spray-dryer scales. To maintain the target droplet size across a tenfold throughput increase, atomization pressures increased from 250 psi at the PSD-1 laboratory scale to more than 1000 psi at the PSD-4 commercial scale.
Although the atomization process can be difficult to model from a first-principles basis due to the complexity of the fluid dynamics involved in droplet formation, empirical correlations have been developed. The spray-solution properties critical to atomization (eg, viscosity, surface tension, density); nozzle geometry (eg, orifice diameter, swirl design); and nozzle operating parameters (eg, nozzle pressure, solution feed rate) can be correlated to predict droplet size.4
While some literature correlations are applicable to SDD spray solutions with viscous polymers, these correlations may need to be validated experimentally or formulation-specific correlations may need to be developed. Correlations for SDD spray solutions can be developed by directly measuring the droplet size using light-scattering or refractive methods such as a phase Doppler particle analysis
(PDPA).5 Active or placebo spray solutions can be sprayed across increasing spray-solution flow rates with increasing nozzle sizes and the resulting droplet-size distributions can be measured. Specific nozzles and nozzle operating parameters can be selected to match droplet size and, thus, SDD particle size across scales.
Figure 3 illustrates the atomization scale-up methodology of matching droplet sizes across scales. Droplet size and resulting SDD particle size for an example HPMCAS spray solution is shown for GEA Niro PSD-1 and PSD-2 spray dryers. The droplet-size correlations can also be linked with correlations of droplet size to particle size and used to evaluate the criticality (and sensitivity) of spray-solution and nozzle operating parameters. In this way, a functional atomization operating space and acceptable SDD particle variance can be defined.
DROPLET DRYING
After the droplet has been formed, drying or solvent evaporation defines the physical state, morphology, density, and residual-solvent content of the SDD.6 Similar to atomization scale-up, the primary goal during scale-up of the thermodynamic parameters is typically to match the drying kinetics or evaporation rate at which the particle forms and, thus, the physical state, morphology, density, and residual-solvent content of the SDD across scales. A methodology for matching droplet drying rates across scales is described below.
Immediately after the droplets are formed, they are quickly contacted with drying gas at the targeted inlet temperature (TIn). Due to evaporative cooling, the droplets rapidly cool from the ingoing solution temperature to the wet-bulb temperature of the solvents, up to the point at which initial solidification or skinning occurs. The timescale for skinning is typically on the order of milliseconds. After skinning, resistance to mass transfer increases dramatically and reduces the evaporation rate since the solvent must diffuse through the skin to the surface for evaporation. The temperature inside the skinned droplet increases to temperatures equivalent to the spray-dryer outlet temperature (TOut).
Figure 4a shows a computational fluid dynamic (CFD) modeling output of the droplet-surface temperature profile in a spray dryer operated at a TIn of 125°C, TOut of 55°C, and a solution temperature (TSoln) of 50°C using an acetone/water spray solvent. As the figure shows, evaporative cooling occurs immediately after the spray solution exits the atomizer, followed by an increase in temperature to TOut. As Figure 4b shows, the SDD drying rate and, thus, SDD CQAs are highly correlated to TOut. Drying rate is also often highly correlated to the relative solvent saturation at the dryer outlet (RSOut).
The droplet drying rate is dictated by the thermodynamic process parameters, which can be related by mass and energy balances at each spray-dryer scale.7 The key thermodynamic parameters and scale-up considerations are summarized in Table 1. The spray-drying process can be operated in a single-pass mode, in which fresh drying gas is introduced to the chamber, or a recycle mode, in which the drying gas and evaporated solvent exiting the chamber are passed through a condenser, reheated, and returned to the drying-gas inlet. Commonly, small-scale dryers operate in single-pass mode, whereas larger spray dryers operate in recycle mode. The effect of the solvent vapor in the recycled drying-gas stream must be considered since it can impact drying rate.7 Differences in heat loss to the ambient environment also must be considered across scales, but generally heat loss becomes less significant as the scale increases.3
The mass and energy balance (ie, thermodynamic model) can be used to predict operating parameters at each scale. Dryer parameters can be selected to produce similar TOut and/or RSOut values and, thus, similar droplet drying rates across scales. A graphical representation of the thermodynamic model is shown in Figure 5a. Figure 5b shows an example operating space for a specific SDD, with example formulation-specific operating-space constraints. Specific operating parameters can be selected within the operating space to match TOut and/or RSOut.
In addition to matching TOut and/or RSOut, a scale factor may also be required to account for differences in droplet and drying-gas mixing efficiencies across scales.
Solution flow rate and plume density increase at larger scales, whereas droplet-to-gas mixing volumes remain relatively unchanged, resulting in decreased mixing efficiencies and slower droplet drying rates at larger scales. Figure 6 shows high-speed camera images focused on the atomizer spray plumes for PSD-1, PSD-2, and PSD-5 spray dryers with acetone-based spray solutions.
Decreased drying rates due to the droplet and drying-gas mixing efficiencies can affect SDD CQAs upon scale-up, but can be easily compensated for by adjusting the process parameters. Scale factors can be developed to define appropriate spray-drying parameters to account for the slower droplet drying. Aided by computational models, dimensionless terms that relate mass- and heat-transport phenomena in and out of the droplets during the drying process are used to relate drying rates across scales.
Figure 7 shows an example of using an adapted dimensionless Damköhler number multiplied by TOut (in Kelvin) as a scale factor. The adapted Damköhler number is calculated as a ratio of reaction rate (evaporation rate, in this case) to convective mixing rate (droplet and gas mixing rate, in this case). The evaporation rate can be calculated using macroscopic thermodynamic process parameters and the mixing rate can be obtained from CFD outputs. In Figure 7a, different correlations are seen with various bulk density and TOut values for three spray-dryer scales, and in Figure 7b, the scale factor is applied to better correlate SDD bulk density across scales.
First-principle thermodynamic processing maps, computer models, and scale correlations are used in parallel to understand and match droplet drying rates across scales. Additionally, these same principles enhance understanding of the operating space and can be coupled with small-scale experiments to further derisk formulation- and process-related constraints. Small-scale experiments can be used to evaluate the predicted commercial operating parameters and can confirm computer-generated or virtual simulations.8 Common examples of small-scale experiments include purposeful variation of SDD CQAs or processing at ranges outside of normal variability to challenge the process and determine the effects on SDD CQAs.
SUMMARY
Use of spray-drying to manufacture amorphous dispersions in the pharmaceutical industry is likely to continue to grow rapidly as the number of compounds that present oral solubilization challenges continues to increase. Efficient scale-up of spray-drying processes is made possible by deep understanding of the spray-drying physical situation. Off-line tools such as droplet-sizing instruments, computer simulations, thermodynamic models, and scale correlations can be used in a systematic manner to identify commercial process parameters and operating spaces without the need for extensive costly experimental runs at commercial scale.
REFERENCES
1. Bloom CJ, Lyon DK. Formulation selection: amorphous dispersions & other tools for drug discovery formulation support. Drug Dev. Delivery. 2013;13(3):32-37.
2. Baumann JM, Dobry D, Ray RJ. Formulation development – amorphous dispersion formulation development: phase- appropriate integrated approaches to optimizing performance, manufacturability, stability & dosage form. Drug Development & Delivery. 2013;13(6):30-36.
3. Marco G, Joao V, Filipe G. Scale-up methodology for pharmaceutical spray-drying. Chemistry Today, 2010; 10(4):18-22.
4. Lefebvre AH. Atomization and sprays. New York: Hemisphere Publishing Corporation; 1989.
5. TSI Incorporated website; www.tsi.com.
6. Vehring R, Foss WR, Lechuga-Ballesteros D. Particle formation in spray-drying. J Aerosol Sci. 2007;38:728-46.
7. Dobry D, Settell DM, Baumann JM, Ray RJ, Graham LJ, Beyerinck RA. A model-based methodology for spray-drying process development, J Pharm Innovat, 2009; 4(3):133-142.
8. International Conference for Harmonisation, Guidance for Industry, Q8(R2) Pharmaceutical Development, November 2009.
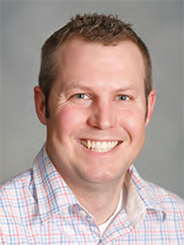
Devon DuBose is a Senior Research Engineer at Bend Research Inc. His responsibilities include spray-drying development and scale up for preclinical and clinical projects, and transferring spray-drying processes into a current Good Manufacturing Practice environment. He also has expertise with special spray-drying projects for biologics and inhalation. Mr. DuBose has been with Bend Research since 2007. He graduated with a BS in Chemical Engineering from Oregon State University and is a registered professional engineer in the State of Oregon. Mr. DuBose has three US patents pending.
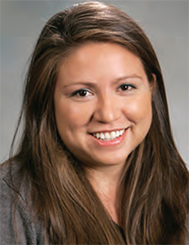
Dana Settell, Vice President, oversees large, late-stage manufacturing and engineering programs at Bend Research. Formerly, she was Director of Business Development. In addition, in a previous position as Director of Operations at the company’s current Good Manufacturing Practice (cGMP) facility, she assisted with the installation, commissioning, start-up, maintenance, validation, and operation of a PSD-2/FSD-4 spray-dryer. Ms. Settell has extensive experience with scaling up drug production from development through commercial production. She was instrumental in the start-up of a $90-million commercial plant in Ireland to manufacture spray-dried dispersion (SDD) technology at the metric ton scale. Ms. Settell earned her BS in Chemical Engineering from the University of Colorado. She holds two US patents and has two publications to her credit.
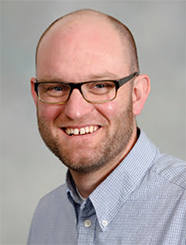
John Baumann is the Director of Spray-Dried Particle Engineering at Bend Research, where he has worked since 2004. Mr. Baumann manages a spray-drying process-development group focused on the preparation of pharmaceutical intermediates and the scale-up and transfer of spray-drying processes from research laboratories to production in a current Good Manufacturing Practice (cGMP) environment. He earned his BS in Bioengineering from Oregon State University.
Total Page Views: 9672