Issue:June 2021
PRECLINICAL TESTING - Expanding Opportunities in Implantable Medical Devices With Optimized Preclinical Studies
INTRODUCTION
Implantable medical devices (IMDs) are increasingly used in established and novel clinical applications. Given such devices’ invasive nature, they are subject to some of the strictest regulations and rigorous pre- and post-market controls. In vivo preclinical models represent a crucial tool to study how these devices function in physiological settings and provide vital supporting evidence for regulatory approval processes. However, standard experimental design and primary endpoints for preclinical studies are often limited, reductionist, and may overlook common and essential clinical aspects of a patient’s condition that could impact device safety and performance.
The following outlines the growing importance of implantable devices in clinical settings and our daily lives, highlighting the current state of preclinical testing and the regulatory barriers faced by device developers. But, as described here, innovative preclinical study design and testing in multiple disease contexts can provide a deeper understanding of device function, enabling more effective product development and more precise insight into clinical effectiveness.
EXPANDING OPPORTUNITIES FOR DEVICES & PATIENTS
IMDs — a subset of medical devices — are used inside the body and include artificial hips, drug-eluting stents, insulin delivery devices, and pacemakers. The market for IMDs is increasing and is expected to reach a global value of $153.8 billion by 2026.1 IMDs have been used for decades in surgical settings to provide life-saving therapies, primarily in orthopedics and cardiology. But more recently, significant interest in IMDs replacing or complementing existing pharmaceutical treatments has been growing in many clinical fields. There are also broader applications for IMDs in personalized medicine, health monitoring, and interfacing directly with physiological systems. Elon Musk’s Neuralink technology, for example, is receiving significant media attention and highlights the scope of the future of IMDs.2
Several developments are facilitating the increased adoption of IMDs in all these contexts. One improvement comes from advances in materials with desirable functional properties that are also safe to use in sensitive locations in the body, such as blood vessels and heart muscle. Plus, the rapid development of wireless and Internet-of-Things technologies over the past decade is facilitating innovation of smaller, smarter implants, and secure wireless technologies and software developments, which expand the functionality of many existing monitoring technologies.
IMD technologies can alleviate patient burdens in many ways. As an example, diabetes, which is increasingly prevalent globally (especially in developed countries, like the US) often relies on patient-driven monitoring and treatment for effective disease management, creating a significant burden to the patient. Such problems could be resolved with effective monitoring and drug-delivering IMDs. Similar devices are set to become increasingly common in treating many conditions and replacing or complementing traditional pharmaceutical approaches
REGULATORY CLASSIFICATION & CONSEQUENCES
The regulatory approval process reflects the different challenges involved in the development of IMDs versus pharmaceuticals. The key differences lie in how regulatory bodies classify IMDs and the resulting consequences for development pipelines. For example, the World Health Organization defines a medical device as: “any instrument, implant, software, or material intended to be used in human beings for a medical purpose, including diagnosis, prevention, monitoring, or treating a disease or injury and for support of the anatomy or of a physiological process.”3
Major regulatory bodies develop classification systems for medical devices that stratify products by risk. The US FDA, for example, uses three categories: low risk (or Class I), which includes IMDs such as elastic bandages and nasal sprays; moderate-risk devices (or Class II), which includes glucose monitoring systems, pregnancy tests, and powered wheelchairs; and high risk (or Class III), which includes IMDs such as drug-eluting stents and pacemakers.4 The regulatory controls required for these classes differ significantly (Figure 1). Implantable and invasive devices are typically higher risk due to invasive procedures, innate immune responses, and material toxicity.5 Consequently, they require stringent pre-market approval (PMA) applications before entering the market. However, approval of these devices can be streamlined by showing equivalence with a marketed device not requiring PMA — termed predicate — via a 510(k).
In contrast to PMA applications, only 10% to 15% of 510(k) applications require clinical data and can rely heavily on preclinical studies to provide the data on the device’s equivalence and performance compared to the predicate device.4 As a result, 510(k) applications offer a quick route-to-market for iterations of existing products, analogous to an abbreviated new drug application (ANDA) for a pharmaceutical.
THE IMPORTANCE OF PRECLINICAL STUDIES
Preclinical studies are extremely valuable in IMD development, from trialing early devices to comprehensive evaluations of efficacy. A study’s design depends on the stage of product development, with endpoints matched to the aims.
Feasibility studies allow initial in vivo tests of device implantation and removal in live animals (under anesthesia), as well as basic assessments of device function and safety. Extensive post-surgical histology is not typically required. Good laboratory practice (GLP) studies, on the other hand, are more involved, with more defined endpoints for safety or functionality. For example, a GLP study of a hemorrhage-control device might involve multiple applications of the device in a surgical setting. A battery of physiological recordings to assess the systemic animal state can be taken before post-surgical histology of the device location to evaluate local tissue damage.
Preclinical studies are used in both 510(k) and PMA applications for IMDs to assess safety and performance. These studies have several significant advantages over clinical trials. In particular, animal studies provide essential information about the physiological impacts of implanting a device important to assessing safety. For example, any implant results in a foreign body response (FBR) from surrounding tissue, which is crucial to understand from a safety and performance perspective (Figure 2).6,7 An FBR is a physiological reaction of the body to foreign material in the tissue. It is a step-wise process of inflammation, wound healing, and potential end-stage fibrosis and scarring.8 If not resolved, excessive fibrosis can result in fibrotic encapsulation of implanted devices, which can impair device integration, long-term functionality, and bioactivity.
Devices implanted in soft tissue or muscle typically create mild fibrosis surrounding the device, altering tissue mechanics and creating a stiffer tissue context for the device.9 Additional local immune responses can cause inflammation, swelling, and bruising around the implantation site as well. These different aspects of FBR can impact device stability, safety, and function, which makes these responses crucial to model and understand. Moreover, the material composition, shape, and rigidity of an IMD can modulate the resulting FBR, which makes these studies part of a feedback loop for iterative design improvements to limit the unwanted response.7,10
Predicting physiological responses to a particular implant in humans is challenging due to significant case-to-case variability based on the device’s specific location in patients. In rats, for example, the microtissue anatomy of different subcutaneous implant sites can alter the FBR.11 Although post-market risk evaluation is an integral part of any IMD development pipeline, there are limitations to the types of endpoints available in human subjects, such as tissue histology. As a result, animal models represent an essential tool for understanding the physiological response to an IMD.
Detailed histopathology from one or more time points after implantation can provide the most accurate assessment of inflammation and fibrosis at the site of implantation.6 Additionally, systemic readouts, such as levels of inflammatory markers in blood or serum, can provide additional data on the inflammatory response, as well as useful functional endpoints for specific devices, such as implantable insulin pumps.
Aside from safety, preclinical studies are also crucial for assessing an IMD’s performance. For instance, Phase 3 drug trials typically involve placebo-controlled studies to determine a drug’s effectiveness versus a control. Equivalent clinical studies for IMDs are impossible due to ethical barriers to unnecessary surgery or implantation of sham devices. As a result, the assessment of a device’s efficacy in controlled conditions or in comparison to other device designs relies entirely on preclinical studies.
As shown, safety and performance studies focus on assessing device performance under routine conditions. For devices used in specific pathological states, preclinical studies tend to replicate this state as simply as possible. For example, vascular-seal devices can be implanted following acute arteriotomy in porcine models, and polymeric drug delivery systems can be implanted in the eye during the various stages of glaucoma in rabbit models. Although informative, these studies do not consider the impact of disease states or localized pathology — such as infection — on device function. This creates a critical knowledge gap in our understanding of how devices perform in realistic clinical settings. Nevertheless, preclinical animal studies can be extended to provide data to guide product development that in vitro or clinical follow-up studies cannot.
A SPOTLIGHT ON CONTINUOUS GLUCOSE MONITORS
Innovative study design and endpoints can help preclinical studies of continuous glucose monitors provide a more robust clinical performance assessment. In the US, approximately 10% of the population suffer from diagnosed or undiagnosed diabetes, a figure that has steadily increased over the past 20 years.12 Standards of care, like finger prick tests and insulin injections, have several limitations, including reliance on patient adherence, regular invasive procedures, and patient-driven treatment. Continuous glucose monitoring (CGM) devices are small implants placed under the skin that regularly report blood glucose levels to the user via a software application. In 2018, Senseonics’ Eversense became the first implantable CGM to gain FDA approval, coinciding with the approval of the interoperable Dexcom G6.13,14 Given the regulatory barriers for breakthrough IMDs, these milestones will likely lead to more devices entering the market over the coming decade.
There are several well-established preclinical models for diabetes in the assessment of CGMs. Figure 3 illustrates the basic study design for testing the primary functional endpoint — blood glucose reading — in a chemically-induced model of diabetes in minipigs.15 These studies allow a direct comparison of blood glucose with the estimated reading provided by the implant in diabetic and control animals, allowing an in vivo evaluation of a device’s performance across a wide range of blood glucose values.
Experiments like these can be combined with follow-up histological assessments of the implant area. For example, a recent study in a rodent model of diabetes found a positive correlation between collagen content at the implant site (a readout of fibrosis) and delay in CGM readings.16 That type of study provides a useful way to understand the underlying causes of the poor performance of CGMs in vivo.
Importantly though, diabetes is a metabolic disease often associated with other comorbidities, including obesity, lung disease, and liver disease. Indeed, smokers are 30% to 40% more likely to develop type 2 diabetes, and non-alcoholic fatty liver disease (NAFLD) increases the risk of type 2 diabetes by two- to three-fold.17,18 These conditions are associated with broad systemic changes to metabolism and immune response and represent a common but distinct physiological context within which CGMs must function properly. Conditions such as NAFLD have established preclinical models, like the Amylin liver NASH (ALMN) diet, which leads to progressive accumulation of fat in the liver, fibrosis, and eventually cirrhosis. These models offer an ideal system for testing the performance of CGM devices under a secondary, clinically relevant disease context. This type of data could lead to device designs that are more universally reliable or to stratify patients to offer specialized devices for those with relevant comorbidities.
THE ROUTE TO SAFER, MORE EFFECTIVE IMDS
Preclinical studies make up a vital stage in the development of IMDs. Established disease models and study designs enable effective assessments of safety and efficacy. However, these models can be further augmented to provide far greater insight into the function of these devices in real clinical settings, namely by combining disease models and correlating device performance with secondary endpoints. Given the growing importance of IMDs in the clinic, and our daily lives, the development of this preclinical approach is more critical than ever.
REFERENCES
- Implantable Medical Devices Market Acumen Research and Consulting. https://www.acumenresearchandconsulting.com/implantable-medical-devices-market. Accessed October 27, 2020.
- Markoff J. Elon Musk’s Neuralink Wants ‘Sewing Machine-Like’ Robots to Wire Brains to the Internet. The New York Times. https://www.nytimes.com/2019/07/16/technology/neuralink-elon-musk.html?searchResultPosition=1. Published July 16, 2020. Accessed October 27, 2020.
- Global Atlas of medical devices. World Health Organization. https://www.who.int/medical_devices/publications/global_atlas_meddev2017/en/. Published 2017. Accessed October 27, 2020.
- Overview of Medical Device Classification and Reclassification. US Food & Drug Administration. https://www.fda.gov/about-fda/cdrh-transparency/overview-medical-device-classification-and-reclassification. Current as of December 19, 2017. Accessed October 27, 2020.
- Implants and prosthetics. FDA. https://www.fda.gov/medical-devices/products-and-medical-procedures/implants-and-prosthetics. Updated September 30, 2019. Accessed October 27, 2020.
- Anderson JM, Rodriguez A, Chang DT. Foreign body reaction to biomaterials. Semin Immunol. 2009; April; 20(2):86-100.
- Veiseh O, Doloff JC, Ma M, et al. Size- and shape-dependent foreign body immune response to materials implanted in rodents and non-human primates. Nat Mater. 2015; 14(6):643-651.
- Dondossola E, Holzapfel BM, Alexander S, et al. Examination of the foreign body response to biomaterials by nonlinear intravital microscopy. Nat Biomed Eng. 2016; 1:0007.
- Doloff JC, Veiseh O, Vegas AJ, et al. Colony stimulating factor-1 receptor is a central component of the foreign body response to biomaterial implants in rodents and non-human primates. Nat Mater. 2017; 16(6):671-680.
- Moshayedi P, Ng G, Kwok JCF, et al. The relationship between glial cell mechanosensitivity and foreign body reactions in the central nervous system. Biomaterials. 2014; 35(13):3919-3925.
- Picha GJ, Drake RF. Pillared-surface microstructure and soft-tissue implants: effect of implant site and fixation. J Biomed Mater Res. 1996; 30(3):305-312.
- National Diabetes Statistics Report, 2020. US Department of Health and Human Services. https://www.cdc.gov/diabetes/pdfs/data/statistics/national-diabetes-statistics-report.pdf. Accessed October 27, 2020.
- Eversense Continuous Glucose Monitoring System – P160048/S006. US Food & Drug Administration. https://www.fda.gov/medical-devices/recently-approved-devices/eversense-continuous-glucose-monitoring-system-p160048s006. Current as of June 6, 2019. Accessed October 27, 2020.
- FDA authorizes first fully interoperable continuous glucose monitoring system, streamlines review pathway for similar devices. US Food & Drug Administration. https://www.fda.gov/news-events/press-announcements/fda-authorizes-first-fully-interoperable-continuous-glucose-monitoring-system-streamlines-review. Published March 27, 2018. Accessed October 27, 2020.
- Strauss A, Tiurbe A, Chodnevskaja I, et al. Use of the continuous glucose monitoring system in Goettingen minipigs, with a special focus on the evaluation of insulin-dependent diabetes. Trans Proc. 2008; 40(2):536-539.
- McClatchey PM, McClain ES, Williams IM, et al. Fibrotic encapsulation is the dominant source of continuous glucose monitor delays. Diabetes. 2019; 68(10):1892-1901.
- 2014 Surgeon General’s Report: The Health Consequences of Smoking—50 Years of Progress. U.S. Centers for Disease Control and Prevention. https://www.cdc.gov/tobacco/data_statistics/sgr/50th-anniversary/index.htm#report. Accessed October 27, 2020.
- Gastaldelli A, Cuis K. From NASH to diabetes and from diabetes to NASH: mechanisms and treatment options. JHEP Reports. 2019; 1(4):312-328.
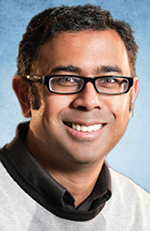
Jaleel Shujath is Vice President, Marketing and Content, at Absorption Systems, a Pharmaron Company. Before joining Absorption Systems, he defined and marketed technology solutions to the life sciences sector, including GE Healthcare, PBL Assay Science, ATCC, and OpenText. He is a Six Sigma Green Belt and a member of the Drug Information Association, Parenteral Drug Association, International Society of Pharmaceutical Engineers, and the Editorial Board of the American Pharmaceutical Review.
Total Page Views: 4874