LIPID NANOPARTICLES - EnCore(TM): Facilitating Delivery of RNAi Therapeutics to Treat Cancer
INTRODUCTION
Throughout the past 3 decades, human genetic data and preclinical models of cancer have facilitated identification of several oncogenes, such as MYC, CTNNB1, KRAS, and BCL2, as well-validated targets for new anticancer therapies. Conventional therapeutic modalities (eg, small molecules and monoclonal antibodies) offer only indirect and thus far inadequate solutions for targeting these oncogenes. For example, small-molecule delivery platforms would target posttranslational modification or regulation of RAS family oncogenes, as opposed to directly inhibiting the function of these proteins. Although indirect MYC inhibitors, such as those targeting BET bromodomain proteins and cyclin-dependent kinase 7 (CDK7), have entered early clinical development, these agents are known to inhibit expression of many genes in addition to MYC, and therefore may have unwanted pharmacological activity in patients.1,2 Given the failure of such indirect approaches to offer therapeutic benefit in the past, as well as the uncertainty whether they will ultimately be successful, their well-validated oncogenic targets were heretofore considered “undruggable.”
The advent of RNA interference (RNAi)-based therapy offers the ability to home in on these targets directly. By enabling targeting at the messenger RNA (mRNA) level, as opposed to the protein level, RNAi allows treatment to focus on any expressed RNA in the genome, conferring advantages of potency and specificity through direct targeting of oncogenes. With RNAi, therapy can be directed at the transcriptional component of the tumor cell, including mRNAs, which encode proteins, as well as non-coding RNAs. Indeed, the power of RNAi lies in “druggability”: the treatment plan can aim at any significant tumorigenic or tumor-related cancer target, yielding therapeutic benefits.
Prior to the advent of therapeutic RNAi programs, most small nucleic acid drug discovery programs relied on RNase Hactive antisense oligonucleotides (ASOs). These ASOs, composed of single-stranded, chemically modified DNA and RNA, enabled the targeting of any cellular RNA for destruction of the RNase H enzyme. The process relied on hijacking the RNase H enzyme to mediate the effects of antisense therapeutics. Unfortunately, the antisense field suffered from the limited number of chemistries that could be applied to the therapeutic platform, while maintaining potency and tolerability, as well as from various pharmaceutical delivery challenges. However, the research, manufacturing, and clinical advances made during the pursuit of antisense therapeutics have greatly benefited the clinical application of RNAi.
The RNAi approach co-opts some functions of the natural RNA-induced gene-silencing pathway, using highly chemically modified double-stranded oligonucleotides to create a therapeutic platform that is chemically and structurally flexible. RNAi works via an efficient catalytic mechanism, yielding a high level of intrinsic potency. With such inherent properties, RNAi has the potential to achieve therapeutic success against previously undruggable oncogenic targets.
Nevertheless, efficient delivery of RNAi therapeutics to tumors can be challenging. One key challenge lies in the inherent instability of RNA caused, in part, by our natural defenses against potential viruses.
If an RNAi trigger is administered intravenously without chemical stabilization or encapsulation within a nanoparticle, it will be degraded by nucleases in the blood and other tissues in a matter of minutes. Additionally, whereas most conventional small molecular drugs are lipophilic, enabling their permeability across cell membranes, RNAi therapeutics are much larger and membrane-impermeable and therefore require a delivery solution. Even when chemically stable, RNAi molecules cannot be dosed systemically without incorporating delivery technology due to their molecular properties. In addition, because they are below the glomerular filtration size limit (approximately 5 nanometers), RNAi therapeutics are excreted through the kidney in minutes unless they are engineered to bypass this effect.3 Such rapid excretion can compromise these molecules’ tumor bioavailability.
Throughout the past decade, multiple research organizations have employed a delivery technology by which a small-molecule ligand, called Nacetylgalactosamine (GalNAc), is attached chemically to the RNAi therapeutic to mediate delivery of nucleic acids to the liver. This approach, which Dicerna Pharmaceuticals, Inc. is using in its DsiRNA-EX conjugate platform, is designed to direct the therapeutic payload to liver cells, where receptors on the hepatocytes recognize and bind to the ligand, pulling it out of the bloodstream. Unfortunately, despite many attempts, this ligand-mediated approach is not useful for delivery to tumors; to date, no ligand-receptor system expressed universally by tumors has been discovered that would enable this type of direct targeting with sufficient specificity. However, nanoparticle technology has the potential to enable tumor bioavailability even in the absence of a targeting ligand.
LIPID NANOPARTICLES: THE RNAi DELIVERY SOLUTION FOR TUMORS
Lipid nanoparticles (LNPs) offer a three-part RNAi delivery solution. The first is encapsulation of the active pharmaceutical ingredient (API), which in this case, is the RNAi therapeutic. Encapsulation within LNPs endows the API with pharmaceutical properties that enable delivery to the site of action. The LNPs can protect the RNAi therapeutic from degradation, rapid excretion, and liver clearance. Encapsulation also facilitates tumor bioavailability, due to the achievement of a plasma half-life of sufficient time to enable uptake in the tumor. With LNPs, tumor uptake may occur more efficiently than in other tissues because of the leaky vasculature in the tumor environment (an effect described in the field as “enhanced permeability and retention,” or EPR). Although the molecular mechanism of LNP uptake into tumor cells is not fully elucidated, it is known to be very different from the mechanism of LNP uptake in the liver.
The second part, penetration of the tumor, is considered a potential advantage of LNP technology. Certain LNPs enable passage of the RNAi trigger through the vascular layer of the tumor to achieve extravasation into the tumor parenchyma. In other words, the LNP not only gets through the first layer of tumor cells, but it is eventually distributed throughout the tumor, homogeneously.
Internalization into the tumor cell is the third step in LNP-based delivery. This property enables trafficking through the tumor cell so that the RNAi trigger eventually reaches the cytosol of the cell, which is where the RNA-induced silencing complex (RISC) – the endogenous RNAi machinery – is located. Engagement and activation of the RNAi molecule within RISC triggers silencing of the target mRNA.
The utility of previous LNP technologies for tumor-directed delivery was limited by the fact that the LNPs either did not accumulate in the tumor at a high enough concentration, or conversely, accumulated in the liver at a level that resulted in unacceptable toxicity. Those previous platforms, which almost invariably arose from liver-directed LNP programs that were retrofitted for tumor delivery, did not mediate efficient delivery of RNAi into the cytoplasm of tumor cells. All liposomes have some degree of filtration out of the liver (which itself is designed to filter out particles), compromising their ability to deliver a therapeutic payload to the tumor. We have also learned through experimentation that the parameters that drive delivery to liver tumors differ greatly from those driving delivery to normal liver cells.
Another limitation of previous LNP technologies is that the liposomes were originally developed as hollow spheres containing a volume of water (ie, an entrapped aqueous space). Indeed, early publications included illustrations depicting free RNA floating inside a liposome “balloon.” From a chemistry and physics perspective, that theoretical structure was utterly incompatible with the particle components: the structure incorporated a highly negatively charged RNA payload with pencil-like rigidity, mixed with positively charged lipids.
ENCORETM LNP TECHNOLOGY: OPTIMIZING TUMOR-DIRECTED DELIVERY
Dicerna’s EnCoreTM LNPs have been designed and optimized for delivering RNAi therapeutics to tumors. All optimization work was performed in tumors instead of first achieving maximum activity in the liver and then making marginal modifications to achieve tumor delivery. This approach is more expensive and labor-intensive because pharmacology models are required for tumor delivery, but it has yielded preclinical data demonstrating knockdown of key oncogenes.4,5
Development of the EnCore LNP technology started by taking the screening approach of particle delivery efficiency and RNAi activity, measuring target knockdown in tumor tissue. After evaluating several “off-the-shelf” single-stranded antisense delivery platforms, Dicerna concluded that none would be functional for double-stranded RNAi. Based on that evaluation, the company decided not to use mouse liver as a surrogate tissue for tumor delivery. Although widely available, inexpensive, and easy to deliver to the liver, normal whole animal tissue is not comparable to tumor tissue. Dicerna therefore decided to focus on a different target tissue with completely different structural, biochemical, and surface properties not comparable to any normal organ.
Another key difference of EnCore LNPs is that their LNP structure is solid, not hollow. They contain a solid core of positively charged lipid, with RNA inside. The well-controlled aggregate makes consistently shaped, solid spheres. The core’s purpose is to bind RNA efficiently, without wasting payload, and to protect it from the environment (ie, during the manufacturing process as well as in vivo).
Other LNP manufacturing approaches for delivering RNA mix all components in a single step. Every component of the particle must solve all independent variables simultaneously: spontaneous aggregation; organization into a sphere that is mechanically and chemically stable; administration into a vein; mediation of biodistribution through the blood; accumulation in target tissue; and delivery of the payload to the cytoplasm. If the particle has poor stability in a vial while stored in the pharmacy, and if any component is tweaked, that variable change ripples through the entire process, creating many challenges.
To overcome such mechanical and initial manufacturing challenges, Dicerna divided the LNP development process into two discrete elements to achieve in vivo pharmaceutical functions: an RNA lipid core and the addition of a thick layer of additional lipids surrounding the core surface. The additional lipids were selected not only for their mechanical properties or manufacturability in loading RNA efficiently, but for biodistribution, specific tissue uptake, and their ability to transfect or mediate delivery of RNA into the cytoplasm of cells. Hence the EnCore name, a portmanteau of Envelope and Core.
VIABILITY OF MYC AS A THERAPEUTIC TARGET
As one of the first discovered oncogenes, MYC is possibly the single-most well-validated oncogenic target, and it remains one of the most extensively studied. Recent mechanistic insights have sparked a resurgence of interest in MYC. Although it has been known for approximately 30 years as causal for tumorigenesis and tumor maintenance, new functions of MYC are constantly being unraveled. In terms of human genetics, a growing body of evidence suggests MYC is involved in >50% of all human tumors.6 Indeed, its targetability appears to be near universal: the more MYC is studied, the more it appears to be upstream of many of Hanahan and Weinberg’s “hallmarks of cancer.”7
Preclinical studies show that DCRMYC, an RNAi therapeutic delivered via EnCore LNPs, inhibited gene transcript activity and reduced tumor volume in multiple mouse models of cancer. Interim results from an ongoing Phase 1 dose-escalation study of DCR-MYC, presented at the 2015 ASCO annual meeting, demonstrated good tolerability and early signs of clinical anti-tumor activity and metabolic responses across various dose levels in patients with advanced solid tumors, multiple myeloma, and lymphoma, supporting early validation of MYC as a therapeutic target.8
The available DCR-MYC data suggest that RNAi may offer a novel and viable strategy for directly targeting MYC. Preclinical models suggest that effective delivery of the MYC RNAi trigger results in significant mRNA knockdown, functional suppression of MYC protein, and by extension, potential therapeutic benefit. For those reasons, Dicerna selected MYC as the oncogenic target for its first EnCore clinical development program.
POTENTIAL FUTURE APPLICATIONS
EnCore LNPs can be used to deliver other RNAi payloads to tumors. For example beta-catenin is another well-characterized, well-validated oncogenic target with abundant human genetic and preclinical evidence.9-11 Dicerna is developing a second-generation EnCore LNP that appears to hold promise for delivering beta-catenin Dicer substrate short-interfering RNA (DsiRNA) molecules to achieve functional knockdown in many tumor types.
The modular nature of the EnCore LNP technology is one of its strengths. With a delivery platform that can mediate delivery to various tumor types, Dicerna can “mix and match” EnCore LNPs with different RNAi payloads. When fully realized, matching of payload to tumor type may therefore potentiate a tailored approach to treatment of genetically defined cancers.
To view this issue and all back issues online, please visit www.drug-dev.com.
REFERENCES
1. Fu LL, Tian M, Li JJ, Huang J, et al. Inhibition of BET bromodomains as a therapeutic strategy for cancer drug discovery. Oncotarget. 2015;6(8):5501-5516.
2. Christensen CL, Kwiatkowski N, Abraham BJ, et al. Targeting transcriptional addictions in small cell lung cancer with a covalent CDK7 inhibitor. Cancer Cell. 2014;26(6):909-922.
3. Gao S, Dagnaes-Hansen F, Nielsen EJB, et al. The effect of chemical modification and nanoparticle formulation on stability and biodistribution of siRNA in mice. Mol Ther. 2009;17(7):1225-1233.
4. Dudek H, Wong DH, Arvan R, et al. Knockdown of beta-catenin with Dicer-substrate siRNAs reduces liver tumor burden in vivo. Mol Ther. 2014;22(1):92-101.
5. Abrams MT. Advances in delivery of Dicer substrate siRNAs (DsiRNAs) to tumors. Presented at Cold Spring Harbor Laboratory RNAi and Oligonucleotide Therapeutics Conference, April 10, 2015.
Available at: http://files.shareholder.com/downloads/AMDA-2IH3D0/108972646x0x830203/72c6660a-5032-4cae-a67d-8f3a9d8b7929/40715CSHL2015v2.pdf. Accessed May 29, 2015.
6. Tansey WP. Mammalian MYC proteins and cancer. New J Sci. 2014;2014:1-27.
7. Hanahan D, Weinberg RA. The hallmarks of cancer. Cell. 2000;7(100):57-70.
8. Tolcher AW, Papadopoulos KP, Patnaik A, et al. Safety and activity of DCR-MYC, a first-in-class Dicer-substrate small interfering RNA (DsiRNA) targeting MYC, in a phase I study in patients with advanced solid tumors.
J Clin Oncol. 2015;33(suppl):abstr 11006.
9. Takigawa Y, Brown AMC. Wnt signaling in liver cancer. Curr Drug Targets. 2008;9(11):1013-1024.
10. Calvisi DF. Molecularly targeted therapies for human hepatocellular carcinoma: should we start from beta-catenin inhibition? J Hepatol. 2015;62(2):257-259.
11. Sebio A, Kahn M, Lenz HJ. The potential of targeting Wnt/beta-catenin in colon cancer. Expert Opin Ther Targets. 2014;18(6):611-615.
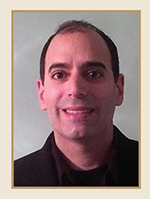
Dr. Marc T. Abrams has served as Senior Director, Preclinical Development at Dicerna since January 2014. He works with his team and colleagues on advancing RNAi platform technologies, developing preclinical drug candidates for specific therapeutic areas, and clinical translation of biomarkers. From 1997-2013, Dr. Abrams has worked at Merck and Co., Inc. in numerous R&D roles, including Research Fellow, Staff Biochemist – Oncology, Senior Research Biochemist – Oncology, and Associate Director – RNA Therapeutics. Dr. Abrams earned his BS in Biotechnology from Drexel University, his MS in Biology from the University of Rochester, and his PhD in Biochemistry from Thomas Jefferson University. He has authored or co-authored 13 peer-reviewed publications in the RNAi field.
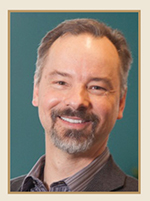
Dr. Bob D. Brown joined Dicerna Pharmaceuticals as Chief Scientific Officer and Senior Vice President (SVP), Research in 2008. He leads the discovery and non-clinical development of RNAi-based therapies, including an agent targeting the MYC oncogene, which entered clinical trials in 2014. Prior to joining Dicerna, Dr. Brown was the Vice President of Research and Technology at Genta, and he worked on more than 75 issued patents and patent applications. Dr. Brown works directly with clinicians and study investigators on trial design, execution and interpretation of results. He earned his PhD in Molecular Biology from the University of California, Berkeley, and his BS degrees in Chemistry and Biology from the University of Washington.
Total Page Views: 4639