Issue:September 2018
LIPID-BASED DELIVERY - Advanced Lipid-Based Drug Delivery Systems: Solid Lipid Nanoparticles & Nanostructured Lipid Carriers
INTRODUCTION
The utility of functional lipids in enhancing the dissolution and bioavailability of poorly water-soluble actives is well known. The use of lipid-based drug delivery systems in pharmaceutical product development is increasing due to the versatility of functional lipid excipients and the compatibility of these excipients with liquid, semi-solid, and solid pharmaceutical dosage forms.1 In addition to solubility and bioavailability enhancement, functional lipids have been employed in a multitude of broad-based excipient applications, including matrix and encapsulated sustained release, tablet lubrication, tablet dry binding, and as pharmaceutical unit operation processing aids.2-5 Although the applications of functional lipids as excipients and drug delivery systems for the pharmaceutical industry has been understood for quite some time, novel features of functional lipids continue to be discovered and employed in ever-emerging drug delivery systems. Two examples of innovative lipid-based nanoparticle drug delivery technologies are solid lipid nanoparticles (SLNs) and nanostructured lipid carriers (NLCs). SLN and NLC formulations maintain the enhanced solubility benefits of traditional liquid colloidal carrier drug delivery systems, but provide several additional benefits, such as increased chemical stability for the Active Pharmaceutical Ingredient (API), potential for sustained release, targeted delivery, and lymphatic delivery, which allows for the avoidance of first-pass metabolism as well as lymphatic targeting.6
SOLID LIPID NANOPARTICLES (SLNS)
SLNs are solid particles of functional lipids typically formulated with fatty acids, monoglycerides, diglycerides, and triglycerides as carrier materials. Additionally, surfactants, such as macrogolglycerides, polaxamers, polysorbates, or ethoxylated castor oils, can be employed as emulsifying agents in the formulations. SLNs are typically formulated with a single high-purity lipid as the primary carrier material. SLNs can be formulated by numerous technologies, including high-shear homogenization (including both hot and cold methodologies), ultrasonication, solvent emulsification/evaporation, micro-emulsion precipitation, supercritical fluid processing, spray-drying, and the double emulsion method.7 There are numerous advantages to employing SLN drug delivery systems, including controlled release, targeted release, increased stability, increased carrier capacity, ability to carry both hydrophobic and hydrophilic actives, reduced systemic toxicity, non-solvent-based manufacturing methods, ease of manufacturability, lower cost formulation materials, and ease of regulatory approval.8
NANOSTRUCTURED LIPID CARRIERS (NLCS)
NLCs are a formulation extension of SLNs in which the NLC lipid particle carrier matrix is composed of multiple lipids. The most common type of NLC matrix is a mixture of a solid lipid with chemically different liquid lipids. The combination of a solid lipid with liquid lipid(s) results in areas of carrier matrix inconsistency, which provides for a series of advantages for NLCs over typical single matrix material SLNs.9 NLCs provide for higher active loading than SLNs, due to the presence of liquid carrier materials, which have a higher solvent capacity in the finished NLC. This increased solvent capacity is due to additional active incorporation space created through the generation of crystal imperfections due to the presence of liquid lipid, in the highly ordered crystal structure of the solid lipid material.10 NLCs provide for a biphasic drug-release pattern, which results from a rapid release of active from the liquid phase of the NLC, with a comparatively slower release of the API from the solid lipid portion of the NLC. This release pattern can be modulated by adjusting the proportions of the liquid and solid lipid carriers within the NLC.11 NLCs also provide for long-term active stability during storage, as the presence of liquid lipid in the carrier particle reduces the amount of active crystallization that will occur.12 NLC formulations require less surfactant than liquid emulsion systems, allowing for greater active loading.1
NANOLIPID MANUFACTURING & CHARACTERIZATION
Hot Homogenization
Hot homogenization is a manufacturing technique carried out at temperatures above the melt point of the lipid(s). A pre-emulsion of the active-loaded lipid melt and an aqueous emulsifier is generated by high-shear mixing. Typically, more than one pass is required through the high-shear homogenizer. It should be noted that increasing homogenization leads to an increase in nanolipid particle size due to particle coalescence. Typically, lower nanolipid particle sizes are obtained at higher processing temperatures due to reduced viscosity of the lipid phase.14
Cold Homogenization
Cold homogenization is carried out on solid lipids. Adequate temperature control has to be in place during this process to ensure the lipid does not melt. Cold homogenization can solve issues faced during hot homogenization, such as heat degradation of the active, partitioning and loss of the active to the aqueous phase, and unwanted polymorphic transitions. Active is dispersed in the lipid melt, which is cooled rapidly. The cooled melt is size reduced by employing a milling operation. Subsequently, the milled particles are dispersed in a chilled emulsifier solution. This dispersion is subjected to high-pressure homogenization at or below room temperature, to generate the nanoparticles. Cold homogenization typically results in larger particles and broader particle size distributions than hot homogenization.15
Ultrasonication/High-Shear Homegenization
In this method, lipid nanoparticles are generated by high-speed stirring or sonification. This is a method that can generate lipid nanoparticles on lab-scale equipment that is readily available. This dispersion technique does not require solvents or large amounts of surfactant. The melted drug and lipid mixture is added and dispersed in aqueous surfactant and placed under high-shear homogenization or ultrasonification. The emulsion is then cooled to room temperature to form the lipid nanoparticles.16
Solvent Emulsification/Evaporation
In this method, lipid nanoparticles are prepared by precipitating oil/water emulsions.17 The lipophilic material is dissolved in a water-immiscible organic solvent that is emulsified in an aqueous phase. Upon evaporation of the solvent, a nanoparticle lipid dispersion is formed by precipitation of the lipid in the aqueous phase.
Micro-Emulsion Based on SLN Preparations
Manufacturing lipid nanoparticles by this method involves dispersing hot microemulsions in cold water under stirring. The emulsions typically consist of a low-melting fatty acid, an emulsifier, co-emulsifiers, and water. The droplet structure is generated by the emulsion globule size, and no additional energy needs to be added to the system to generate submicron particle sizes.18
Supercritical Fluid
In this method, solutions of supercritical CO2, active, and lipid are rapidly expanded into an aqueous phase containing surfactant and then sonicated and lyophilized to obtain the lipid nanoparticles.19 A major advantage of this system is that no organic solvents are required.20
Spray Drying
Manufacturing lipid nanoparticles by spray drying is a one-step process that converts a liquid feed of organic solvent solution, in which the lipid-active mixture is dissolved, which is atomized by spraying. This spray atomization contacts a hot gas that results in the evaporation of the solvent, forming dried lipid particles. The dried particles are separated from the gas by a cyclone, an electrostatic precipitator, or bag filter.21
Double Emulsion
Lipid nanoparticles are obtained from water/oil/water multiple emulsions employing solvent-in-water emulsion-diffusion techniques. Typically, a hydrophilic active can be dissolved in the aqueous inner phase and consequently carried in the interior of the lipid nanoparticle. The lipid nanoparticle is generated by aqueous dilution of the water/oil/water emulsion.22 This manufacturing technique can readily incorporate both hydrophilic and hydrophobic actives.
Common characteristics evaluated for lipid nanoparticles are particle size and particle size distribution by photon correlation spectroscopy and laser diffraction. Also: zeta potential by zetameter to predict particle agglomeration potential, electron microscopy to determine particle shape, nuclear magnetic resonance to determine particle size and qualitative structure of the particles, x-ray diffraction to determine the degree of crystallinity, and differential scanning calorimetry to determine the nature and speciation of crystallinity within lipid nanoparticles.23,24
DRUG DELIVERY ADVANCEMENTS WITH NANOLIPIDS
Two major drug delivery advancements of SLNs and NLCs are the lymphatic uptake of these materials, as well as the ability of these materials to transport actives across the blood brain barrier (BBB).
The lymphatic system is part of the circulatory system, which is composed of an intricate network of conduits that carry lymph. The primary function of the system is to carry interstitial fluid accumulation back to the circulatory system and to transport immune cells to the lymph nodes.25,26 Per-oral actives incorporated in SLNs and NLCs reach the systemic blood circulation through the intestinal lymphatic system, as opposed to entering the systemic blood circulation through the portal blood. This pathway allows for a reduction in first-pass metabolism and an increased overall bioavailability for the active.27 In addition to intestinal lymphatic uptake, SLNs and NLCs can be delivered by means of other lymphatic pathways, including subcutaneous and pulmonary lymphatic uptake.
Advantages of subcutaneous lymphatic uptake of actives are active accumulation at the site of administration, low clearance, avoidance of first-pass metabolism, sustained release, and increased absorption. Advantages of the pulmonary lymphatic uptake include avoidance of first-pass metabolism, reduced systemic toxicity, reduction in the need for continuous dosing, increased local concentration of active, and direct delivery of the active into less-accessible parts of the lung.28
Darunavir is effective against wildtype and PI-resistant HIV, and has a very low oral bioavailability of 37%. It is formulated with ritonavir to increase its bioavailability to 82%. This low bioavailability is common for oral anti-retrovirals, which typically have low-aqueous solubility, high CYP-mediated metabolism, and are often a substrate for P-glycoprotein efflux.29
In order to study the effect of lipid nanoparticle drug delivery on the bioavailability of darunavir, SLNs composed of darunavir, glyceryl monostearate, glyceryl caprylate, Span 80, and Tween 80 were prepared by high-pressure homogenization and subsequent lyophilization. The darunavir SLNs were evaluated for lymphatic uptake, dissolution, and bioavailability. The in vivo studies were conducted in male Wistar rats. It was observed that the in vitro dissolution of darunavir from SLN was slower and less extensive than the dissolution of darunavir from marketed tablets (Figure 1); in contrast, the bioavailability of darunavir from SLN was significantly higher than the bioavailability from the marketed tablets (Figure 2). Additionally, lymphatic uptake evaluation indicated that the SLN exhibited significant lymphatic absorption of darunavir (Figure 3).30
These results demonstrate the efficacy of darunavir SLNs with regard to both uptake by the lymphatic system and overall increase in bioavailability due to lymphatic absorption of the formulation (Figure 4). These points are further enforced given that the in vitro release of darunavir SLNs was slower and less extensive than marketed tablets, yet the bioavailability was much greater.
The BBB is a group of astrocytes, neurons, and endothelial cells that restrict passage of numerous biological or chemical entities to the brain tissue. It provides significant protection to the brain, but this protection inhibits the delivery of many actives to the brain, and therefore, the BBB represents a significant challenge to drug delivery.31 The most formidable obstacles that impede drug delivery to the brain are the largely impermeable endothelial cells and tight junctions, P-glycoprotein efflux, and enzymatic activity. Both SLNs and NLCs exhibit bioacceptable and biocompatible characteristics compared with polymeric nanoparticles, and they are more readily able to traverse the BBB due to their lipidic nature.32
Lamotrigine (LMT) is a broad spectrum efficacy antiepileptic active. Over-expression of P-glycoprotein in the BBB reduces the passage of LMT across the BBB.33 Due to the limited passage of LMT into the brain, relatively high doses are required to reach therapeutic concentrations. These high doses lead to high plasma concentrations of LMT, which can lead to systemic side effects.
In order to evaluate the effect of NLC on the bioavailability of Lamotrigine, LMT containing NLCs were formulated by solvent evaporation method. The NLC was composed of glycerol monostearate (GMS) as a solid lipid and oleic acid (OA) as a liquid lipid. The GMS, OA, and LMT were dissolved in a mixture of ethanol and acetone (1:1). The solvents were evaporated off with subsequent centrifugation to obtain the final active carrying NLC. Intranasal LMT-NLCs, intranasal LMT solution, and oral LMT solution were evaluated for plasma and brain concentration of LMT. The LMT-NLC and LMT solution were evaluated for in vitro LMT release.34 Additionally, malondialdehyde (MDA) levels and glutathione (GSH) levels were measured to gauge the maximal electric shock (MES) seizure mitigation levels of LMT administered in each respective formulation, as typically MDA levels increase and glutathione levels decrease after MES induction.35 It was observed that the in vitro release of LMT from NLCs was slower and less extensive than the release of LMT from solution (Figure 5). Plasma concentration for LMT-NLCs was lower than plasma concentration for intranasal solution of LMT and roughly equivalent to the plasma concentration of the oral solution of LMT; however, brain tissue concentration was significantly higher for LMT-NLCs than either intranasal solutions or oral solutions of LMT (Figure 6). GSH levels after MES induction were highest in the LMT-NLC formulation, while MDA levels after MES induction were the lowest in the LMT-NLC formulation (Figures 7 & 8).
These results demonstrate the efficacy of LMT-NLCs in enhancing the brain concentration of LMT as compared to intranasal and oral solutions of LMT. The results are more impressive in light of the reduced in vitro dissolution observed for LMT-NLCs, indicating that lipid-based nanoparticles can produce active bioavailability, which is not directly predictable by in vitro dissolution testing.
SUMMARY
Functional lipids have long been employed effectively in pharmaceutical formulations as excipients, solubility enhancers, and processing aids. More recent applications employing functional lipids are focused on targeted drug delivery and overcoming complicated absorption issues to increase bioavailability of actives as well as target actives to specific tissue locations. SLNs and NLCs are effective, easily manufactured drug delivery technologies that allow for a multitude of benefits, including increased active bioavailability, decrease active metabolism and degradation, lymphatic transport of actives, transport of actives across the BBB, extended and biphasic active release, multiple routes of administration, and tissue-targeted active delivery. Increased research and development of these drug delivery platforms will continue to advance therapeutic efficacy and safety for emerging difficult-to-deliver actives.
REFERENCES
- Katja C, et al. Acta Pharm. 2013;63:427. Lipid-based systems as a promising approach for enhancing the bioavailability of poorly water-soluble actives.
- Abouelatta S, et al. Eur J Pharm Biopharm. 2015;89:82. Floating lipid beads for improvement of bioavailability of poorly soluble basic drugs: In-vitro optimization and in-vivo performance in humans.
- Bolhuis C, et al. Drug Dev Ind Pharm. 2008, Oct, 15. Mixing action and evaluation of tablet lubricants in direct compression.
- Onyechi J, Udeala O. Bio-Research. 2012;9(2):787. Auxiliary dry binding properties of some hydrogenated vegetable oils in direct compression tableting.
- Valla C, et al. 2012. WO2012021432A2. Process of manufacturing a stable softgel capsule containing microencapsulated bacteria.
- Khan A, et al. Int J Nanomedicine. 2013;8:2733. Advanced drug delivery to the lymphatic system: lipid-based nanoformulations.
- Chih-Hung L, et al. J Food Drug Anal. 2017;25:219. Recent advances in oral delivery of drugs and bioactive natural products using solid lipid nanoparticles as the carriers.
- Mukherjee S, et al. Indian J Pharm Sci. 2009;71(4):349. Solid lipid nanoparticles: A modern formulation approach in drug delivery system.
- Khan S, et al. Int J Pharm Investig. 2015;5(4):182. Nanostructured lipid carriers: an emerging platform for improving oral bioavailability of lipophilic drugs.
- Zhuang C, et al. Int J Pharm. 2010;394:179. Preparation and characterization of vinpocetine loaded nanostructured lipid carriers (NLC) for improved oral bioavailability.
- Tiwari R, Pathak K. Int J Pharm. 2011;415:232. Nanostructured lipid carrier versus solid lipid nanoparticles of simvastatin: comparative analysis of characteristics, pharmacokinetics, and tissue uptake.
- Maincent M, Muller R. Drug Dev Ind Pharm. 2008;34:1394. Lipid nanoparticles with solid matrix (SLN, NLC, LDC) for oral drug delivery.
- Yang Y, et al. J Colloid Interface Sci. 2014;418:261. The effect of oil type on the aggregation stability on nanostructured lipid carriers.
- Lander R, et al. Biotechnol Prog. 2000;16:80. Gaulin homogenization: a mechanistic study.
- Mullen zur A. PHD Thesis, Free University of Berlin. 1996. Feste Lipid-Nanopartikel mit prolongierter Wirkstoffiberation: Herstellung, Langzeitstabilitat, Charakterisierung, Freisetzungsverhalten und mechanismen.
- Uner M. Pharmazie. 2006;61:375. Preparation, characterization and physio-chemical properties of Solid Lipid Nanoparticles (SLN) and Nanostructured Lipid Carriers (NLC): their benefits as colloidal drug carrier systems.
- Sjostrom B, Bergenstahl B. Int J Pharm. 1992;88:53. Preparation of submicron drug particles in lecithin-stabilized o/w emulsions: I: Model studies of the precipitation of cholesteryl acetate.
- Gasco M. United States Patent No. 1993 US 188837. Method for producing solid lipid microspheres having a narrow size distribution.
- Ahmad I, et al. J Pharm Innov. 2016;11(4):308. Supercritical fluid technology trans-resveratrol SLN for long circulation and improved radioprotection.
- Chen Y, et al. Zhongguo Zhong Yao Za Zhi. 2006;31:376. Preparation of solid lipid nanoparticles loaded with Xionnggui powder-supercritical carbon dioxide fluid extraction and their evaluation in-vitro release.
- Battaglia L, Gallarate M. Expert Opin Drug Deliv. 2012;9(5):497. Lipid nanoparticles: state of the art, new preparation methods and challenges in drug delivery.
- Gallarate M, et al. J Microencapsul. 2009;26(5):394. Preparation of solid lipid nanoparticles from W/O/W emulsions: preliminary studies on insulin encapsulation.
- Meyer E, Heinzelmann H. Surface Science. 1992:99. New York: Springer Verlag. Scanning tunneling microscopy II.
- Drake B, et al. Science. 1989;243:1586. Imaging crystals polymers and process in water with AFM.
- Miteva D, et al. Circ Res. 2010;106:920. Transmural flow modulates cell and fluid transport functions of lymphatic endothelium.
- Iqbal J, Hussain M. Am J Physiol Endocrinol Metab. 2009;296:E1183. Intestinal Lipid Absorption.
- Porter C, et al. Nat Rev Drug Discov. 2007;6:231. Lipid and lipid-based formulations: optimizing the oral delivery of lipophilic drugs.
- Walker J, et al. Alcohol Clin Exp Res. 2009;33:357. Ethanol exposure impairs LPS-induced pulmonary LIX expression: alveolar epithelial cell dysfunction as a consequence of acute intoxication.
- Vermeir M, et al. Drug Metab Dispos. 2009;37:809. Absorption, metabolism, and excretion of darunavir: a new protease inhibitor administered alone and with low dose ritonavir in healthy subjects.
- Bhalekar M, et al. Drug Deliv. 2016;23(7):2581. In-vivo bioavailability and lymphatic uptake evaluation for lipid nanoparticulates of darunavir.
- Pandey P, et al. Tissue Barriers. 2016;(4):1, e1129476-1. Blood brain barrier: an overview on strategies in drug delivery, realistic in-vitro modeling and in vivo live tracking.
- Kaur I, et al. J Control Release. 2008;127:97. Potential of solid lipid nanoparticles in brain targeting.
- Potschka H, et al. Neurosci Lett. 2002;327:173. P-glycoprotein mediated efflux of phenobarbital, lamotrigine, and felbamate at the blood-brain barrier: evidence from microdialysis experiments in rats.
- Alam T, et al. Expert Opin Drug Deliv. 2015;12(2):181. Optimization of nanostructured lipid carriers of lamotrigine for brain delivery: in vitro characterization and in-vivo efficacy in epilepsy.
- Sudha K, et al. Clin Chim Acta. 2001;303:19. Oxidative stress and antioxidants in epilepsy.
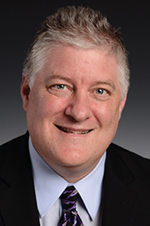
Dr. John K. Tillotson research areas include functional lipids, SEDDS development, and direct-compression tableting. He joined ABITEC Corporation in 2015, and currently serves as the Pharmaceutical Technical Business Director for the Americas, responsible for business development and product technical support in the US, Canada, and Latin America. He has extensive experience in the development of solid oral dosage forms, including the development of multiple direct compression, drug delivery systems, and formulations. He earned his BS in Pharmacy (Ferris State University, MI) and his PhD in Industrial Pharmacy (University of Cincinnati, OH). He can be reached at (614) 429-6464 or jtillotson@abiteccorp.com.
Total Page Views: 11611