Issue:September 2021
GENE THERAPY - Solving the Puzzle: Aligning the Pieces of Gene Therapy & Creating Success for Patients
INTRODUCTION
For a two-word phrase, the term “gene therapy” encompasses diverse and critical elements that are essential for delivering genetic material to target cells in order to treat or cure disease. Each of these elements presents challenges and opportunities for companies developing gene therapies. Each element can be a point of potential failure due to safety concerns, poor targeting of the affected cells types, or insufficient expression to achieve the desired biologic effects. On the flip side, unique and proprietary technologies that can optimize safety and efficacy may enable differentiated approaches to transgene engineering or vector development that provide clinical, regulatory, and competitive benefits. Ultimately, the seamless integration of the understanding of vector biology, genetic engineering, manufacturing, and the unmet patient needs is essential for developing transformative therapies that offer substantial clinical and commercial potential.
When embarking on the development of a novel gene therapy, it is important to keep in mind that the most direct route to the commercial marketplace may not be a straight line. While leveraging existing vectors and promoters might appear to provide an opportunity to reduce development time and risk, the reality is that effective gene therapies must be built from the ground up on a case-by-case basis, if they are to provide optimal performance in the context of a specific disease. This article will review the various elements that must come together in developing safe, effective, and commercially viable viral-based gene therapies.
VIRAL VECTORS
Viral vectors are a foundation of gene therapies because the viruses from which they are derived have evolved over millions of years to enter and deliver genetic material into human cells with high efficiency. Viruses, however, vary in the types of cells they infect, the efficiency with which they enter cells, how long they persist within an infected cell, the size of the genetic payload they can deliver, and the extent to which they activate host immune responses or even cause illness. The ease of vector manufacturing is also highly dependent on viral type.
Each vector offers unique features and benefits that must be carefully paired with the specific disease indication and the desired biologic activity for which it will be used.
For example, adenoviral vectors offer relatively large payload capacity and ease of manufacturing, but also have the potential to trigger robust immune responses that can lead to serious, and in one case, fatal inflammatory responses. The majority of adenoviral subtypes have a tropism for cells in the respiratory system, while others can efficiently transduce renal, intestinal, or ocular cells. The effect of adenoviral vectors is also short lived, which, while potentially beneficial in certain limited circumstances, would require ongoing treatment in the case of genetic or chronic indications, thus aggravating this tendency of problematic immune responses. Lentiviral vectors offer relatively large payload capacity, the ability to treat cells that rapidly turnover, and can be engineered to have tropism for a broad range of target cells. In contrast to adenovirus and adeno-associated virus (AAV) vectors, however, they can integrate into host cell DNA, resulting in potential interference with other important genes and unwanted alteration of transduced cells. Lentiviral vectors also stimulate innate and adaptive immune responses that can limit efficacy and may cause inflammation. AAV vectors have somewhat more limited payload capacity and more complex manufacturing requirements, but multiple serotypes, combined with careful selection of promoters and other DNA regulatory elements, allow for targeting vectors to specific tissues. Additionally, AAV is not known to cause any human disease, which offers potential safety benefits compared with other viral vectors.
Which one, then, to choose? It depends on the therapeutic needs of the disease for which a gene therapy is being developed. For example, AGTC utilizes engineered AAV-based vectors for its inherited retinal disease (IRD) programs, because they can, in most cases, accommodate the size of the genes that need to be delivered and have reduced potential for inflammation compared with adenoviral and lentiviral vectors, which is particularly important for the treatment of ocular diseases in which inflammation may cause interference with visual function and long-term damage. There are a variety of AAV serotypes that can be used to target specific cell types with high transduction efficiency based on the biological need of a given indication, and AAV capsids can also be engineered both for increased transduction efficiency of cells for which they have native tropism, as well as to transduce additional cells types. As an example, AGTC utilizes an engineered AAV vector (AAV-TYF), which has been optimized for transduction of non-human primate photoreceptor cells, for X-linked retinitis pigmentosa (XLRP) and achromatopsia (ACHM).
PROMOTER ELEMENTS
The desired therapeutic effect of a gene therapy also guides promoter selection. Promoters, which are the DNA regulatory sequences that control in which cells a gene is expressed, can be chosen based on tissue specificity, whether they are constitutively active or respond to physiologic signals, and the levels of expression that they drive. Again, the goal is to build a vector that expresses an appropriate level of protein in the target cell and minimizes expression in non-target cells. Given that a single vector may have tropism for multiple tissues, or multiple cell types within an organ system, promoter elements play a key role in targeting expression to specific cell types with greater precision. Bottom line? Capsids and promoters can be combined to maximize expression in multiple cell types or to restrict expression to specific cells depending on the biology of the delivered gene and the targeted disease indication.
For example, AGTC is currently developing AAV-based gene therapies for XLRP and ACHM, both of which are IRDs. AAV-TYF efficiently transduces both rod and cone cells within the retina, but only cone cells play a role in the pathology of ACHM, and expression of the gene used for ACHM therapy in rod cells could be detrimental. Consequently, the ACHM vector utilizes a cone-specific promoter in order to restrict expression to cone cells even if the vector transduces rod cells, which should eliminate safety issues arising from off-target gene expression. In contrast, the XLRP construct uses a promoter that is active in both rod and cone cells, as both cell types contribute to the XLRP disease pathology (Figure 1).
TRANSGENE CONSIDERATIONS
In addition to optimizing delivery and expression to address the molecular basis of disease, the transgene itself plays a critical role in the safety and efficacy of gene therapies. Here again, understanding the biology of a particular indication is essential for defining the parameters that guide transgene selection and engineering. In some cases, therapeutic activity may require a transgene that produces a protein with absolute fidelity to the wild-type protein. In other cases, transgenes may benefit from codon optimization that yields a protein with enhanced expression and/or stability (Figure 2, left). Depending on the therapeutic gene and vector payload capacity, transgenes may also need to be engineered to be smaller but still produce proteins that provide therapeutic benefit (Figure 2, center).
For example, current clinical programs evaluating gene therapy for the treatment of Duchenne muscular dystrophy (DMD) encode smaller versions of the dystrophin protein. This is because the wild-type dystrophin gene, which is one of the largest genes in the human genome, is too large for any of the currently available viral vectors. Consequently, DMD gene therapies use mini- or micro-dystrophin genes that have been engineered to retain critical functions. Although long-term data on the safety and efficacy of this approach have not yet been generated, preliminary evidence suggests that these smaller genes may provide functional benefit to patients with DMD.
Alternatively, dual vector systems may enable delivery of large, multi-domain proteins that cannot be packaged in current viral vector systems. Such systems could enable delivery of larger genes, such as the therapeutic gene for Stargardt disease, ABCA4. This can be achieved by splitting the DNA into two pieces that share an overlapping region at one end, packaging the pieces into separate vectors, and then delivering both vectors to a single cell. Once inside the cell, the overlapping regions in each gene fragment would mediate DNA recombination to create the full-length gene sequence (Figure 2, right). The efficiency of this recombination is highly influenced by the specific sequences chosen, which need to be tested and proven in appropriate animal models. Here again, vector and promoter selection will also be important for ensuring the complete protein is efficiently expressed in target cells.
VECTOR ADMINISTRATION
Other factors beyond the vector components themselves may also play a significant role in the safety, efficacy, and commercial viability of gene therapies. The route of vector administration is one such factor. Gene therapies can be administered via subretinal or intravitreal injection for the treatment of ocular diseases; through the round window of the middle ear for the treatment of otologic diseases; and via injection into the spinal cord or directly into the brain ventricles for the treatment of neurologic diseases. In each of these examples, the route of administration may have an impact on the total dose needed to achieve therapeutic benefit, the ability to target specific cell types or subtypes, and the simplicity versus complexity of administration. Additionally, more complex or invasive administration routes may pose a barrier to patients wishing to undergo therapy, although this would likely be a greater issue for indications in which other effective therapies are available, rather than for diseases in which a gene therapy would be a significant new advancement. Novel routes of administration may also require availability and testing of devices used to deliver gene therapies. Consequently, the route of administration is another important piece of the gene therapy puzzle and should be considered at the earliest stages of development in order to ensure the route and therapy have been optimized for use together.
MANUFACTURING
While the strategic selection of vectors, promoters, and transgenes is essential for the safety and efficacy of gene therapies, robust manufacturing processes are critical for commercial success and broad patient access. Scalable processes that can be performed in bioreactor systems similar to those used for decades to produce a variety of protein and antibody therapies can enable cost-effective manufacturing to meet small rare disease indications, such as IRDs, while providing capacity to support the development of gene therapies for large markets, such as age-related macular degeneration. Bioreactor-based methods also reduce the need for manual tissue culture processes that carry the risk of operator error or contamination.
A key challenge in gene therapy manufacturing is balancing the “need for speed” with the development of processes that meet regulatory requirements and will support commercial use. Many gene therapies are initially developed in academic laboratories using small-scale production methods that are appropriate for preclinical studies and may even have sufficient yield for small Phase 1 trials. These processes are not, however, sufficiently robust for late-stage clinical development or commercial manufacturing. Commercial-scale gene therapy manufacturing will require significant investment in process development, facility build-out, or contract manufacturing. Companies must strike the right balance between investing early in programs that may not warrant continued development and waiting until product candidates near approval and commercialization, which could delay the initiation of late-stage trials.
Gene therapy manufacturing processes must also be designed with high yield for vectors that have the complete gene cassette packaged within them, with limited numbers of empty vectors and with the efficient removal of the residual raw materials, such as host cell proteins and DNA. Since the initiation of its AAV manufacturing efforts, AGTC has significantly increased the productivity of its process, resulting in the current process that offers the potential for a 90-fold reduction in the cost of goods sold. Additionally, the process results in 90% complete capsids and undetectable residual raw material. This is important for enabling optimized dosing by delivering a therapeutic dose with the lowest total viral load possible, and without impurities that could lead to side effects or toxicities.
Vector characterization is another critical element of manufacturing, as it ensures the safety, efficacy, and potency of the finished product. Regulatory agencies put as much emphasis on ensuring the safety, quality, and reproducibility of gene therapies as they do on their clinical safety and efficacy. As an illustrative example, in September 2020, the US FDA requested that Sarepta utilize an additional potency assay for release of the commercial process material prior to dosing in the company’s planned Phase 3 trial of its gene therapy for DMD. This request underscores the importance of vector characterization as an intrinsic part of developing gene therapies.
ASK THE EXPERTS
The safe, effective, and successful assembly of the pieces of the gene therapy puzzle requires one other critical ingredient – disease-specific expertise. As highlighted earlier, the choice of vector, promoter, transgene, and route of administration must be done with an eye toward optimally addressing the biology of a disease that a specific gene therapy is designed to treat. This can only be achieved with insight from those who are truly expert in that disease.
For larger biopharmaceutical companies, such expertise may reside in-house and can be integrated with internal gene therapy research and development efforts. Smaller companies may achieve the same objective through strategic partnerships and collaborations that synergistically utilize complementary expertise and technologies. For AGTC, this has meant establishing collaborations with Bionic Sight and with Otonomy, to develop gene therapies for vision loss and otologic disease, respectively. In each relationship, AGTC provides expertise in gene therapy development while the partner brings expertise in optogenetics (Bionic Sight) or genetic hearing disorders. This allows development of an optimized (Otonomy) vector and administration strategy for each indication that is based on the biology of the disease and desired therapeutic outcome.
A PUZZLE WORTH SOLVING
With diverse factors and options at every step of the continuum, the path for developing gene therapies can be viewed as ridden with multiple hurdles and complexities. Yet a diligent, data-driven, and patient-focused approach to navigating this path offers unique opportunities to truly advance and transform the treatment of serious diseases that have urgent unmet need. With the potential to optimize all aspects of gene therapy development to optimize safety, efficacy, and patient outcomes, we cannot accept anything less. The patients who stand to benefit from successful gene therapy development certainly won’t.
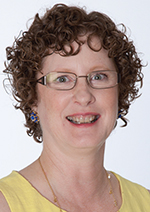
Sue Washer is President and CEO of AGTC and brings a decade of experience in pharmaceutical management and research with Abbott Labs and Eli Lilly & Company and more than 26 years of senior management experience with entrepreneurial firms, including three start-ups. At AGTC, she has secured private and public investments of over $290 million for AGTC, negotiated and closed two major collaborations with top Biotech companies resulting in over $150 million of cash in-flows, and led AGTC in efficiently completing critical scientific milestones. She is an appointed member of the Small Business Capital Formation Advisory Committee for the SEC, Associate Vice President of the ECSGB Board of Bio, and currently serves on the Board of Directors of BIO, and BioFlorida. She earned her undergraduate degree in Biochemistry from Michigan State University and her MBA from the University of Florida, where she was one of the first graduates from the Warrington College of Business Entrepreneurship program.
Total Page Views: 3917