Issue:June 2023
FORMULATION FORUM - PLGA – A Versatile Copolymer for Design & Development of Nanoparticles for Drug Delivery
INTRODUCTION
Poly (lactic-co-glycolic acid) or PLGA, a highly hydrophobic copolymer composed of lactic acid and glycolic acid, has been approved in many drug products and medical devices.1 Throughout the years, since first approved in 1997, PLGA has been a widely studied polymer in the industry because of its compatibility and safety.2 As a homopolymer of two polymerized acidic moieties, one is relatively a hydrophobic lactic acid, and the other is a hydrophilic glycolic acid. PLGA with its unique LA/GA composition ratios and molecular weight provides a good barrier to protect the premature release and degradation of drugs encapsulated in microspheres or nanoparticles. Because of its biocompatibility and biodegradability in nature, it also has been used in several oncology drugs.3
The following will focus on the chemistry, properties, applications, and regulatory aspects of PLGA, and the future trends in the industry, especially those requiring the development of long-acting injectables for the treatment of a variety of rare diseases and for life cycle management.
STRUCTURE OF PLGA
PLGA is synthesized by ring-opening chemistry that follows the copolymerization of a monomer of lactic acid and glycolic acid moieties. PLGA is a polyester in which these moieties are linked together by ester linkages to a linear aliphatic polymer as shown in Figure 1.
Different grades of PLGA are commercially available for pharmaceutical applications as microspheres, nanoparticles, and medical devices. Depending upon the molar ratios of lactic acid and glycolic acid used in the synthesis, the PLGAs used for pharmaceutical applications are composed of 75% lactic acid and 25% glycolic acid and/or 50% each of lactic acid and glycolic acid. PLGA, with its lactic acid and glycolic acid molar ratios of 75:25, 50:50, or 85:15, exists in an amorphous state.4 PLGA (Tg 40°C-60°C) is typically soluble in a wide range of chlorinated methylene chloride chloroform, and hexafluoro isopropanol (HFIP) and non-chlorinated solvents, such as THF, acetone, DMSO, glycofural, ethyl acetate, ethanol, N-methyl pyrrolidone, methyl ethyl ketone, acetonitrile, isosorbid dimethyl ether, dioxane, among others.5 In water, PLGA biodegrades by hydrolysis of its ester linkages. The methyl side group in poly lactic acid moiety makes it relatively more hydrophobic than polyglycolic acid, and hence, lactide rich PLGA copolymers are less hydrophilic and susceptible to degradation by hydrolysis.6 Faster degradation depends on the ratios of the two acids; the higher the lactic acid, the slower the degradation and vice versa. In LA/GA 1:1 copolymer, the degradation is fast, leading to formation of lactic acid and glycolic acid within 3 months. Lactic acid is metabolized in tricarboxylic acid cycle and gets excreted via carbon dioxide and water, whereas, glycolic acid metabolizes the same pathway as lactic acid, and it gets excreted through the kidneys. Glycolic acid is also metabolized into oxalic acid, which may lead to systemic toxicity in the body during implantation or longer circulation of PLGA microspheres.
CHARACTERISTIC & DRUG DELIVERY OF PLGA MICROSPHERES
Physico-chemical properties of PLGA, such as molecular weight, LA:GA copolymer ratios, crystallinity, nature of drug, and its loading, as well as the particle size, morphology and porosity of the nanoparticles may affect the in vitro drug-release characteristics.7 The challenges in utilizing PLGA stems from encapsulating hydrophilic drugs into hydrophobic PLGA. The challenges with reducing burst release and increasing encapsulation efficiency of hydrophilic drugs in PLGA require additional functional hydrophilic layers. To overcome this issue, additional functional layers, like hydrophilic gels/hydrogels around the PLGA core-shell microspheres for sustained release are used. Yu et al demonstrated losartan-loaded PLGA microspheres with gel cores along the exteriors yielded high encapsulation efficiency of water-soluble drugs.8 By selecting 5% gelatin in the inner core, release was more than 30 days compared to 16-day release with water in the inner cores. With 25% Poloxamer 407 as a hydrogel, it showed initial slower release, but as P407 swelled, microcapsules cracked resulting in faster release of drugs. In other cases in which Poloxamer 407 hydrogel can encapsulate small molecules like goserelin, a hydrophilic peptide, and acts as a hydrogel depot at the exterior, PLGA depot encapsulated with the same drug prepared via the double emulsion solvent evaporation method resulted in a combined encapsulation efficiency of >94%. The resulting di-depot structure with PLGA and Poloxamer 407 showed 2-week controlled release, first diffusion of drug through PLGA core followed by diffusion of drug through Poloxamer 407 core.8
PLGA microsphere’s core shells are distinct in nature, and depending upon the usage of these nanoparticles, they may have varying core shapes, internal structures, shell thicknesses, and morphologies and porosities.9 The latter is most explored for designing and increasing drug loading in PLGA microspheres for controlling the delivery by changing the porosity of the PLGA cores. Pore size and porosity of PLGA microspheres with broader surface area and lower density play an important role in faster releasing of drugs.10 A number of porogen agents, such as sodium chloride, ammonium bicarbonate, a tri-block copolymer Poloxamer 407, sodium oleate, gelatin, bovine serum albumin, cyclodextrins, and mineral oils have been investigated for creating porous PLGA microspheres. Porosity can be modified by solvents and polymer concentrations. For example, solvents with lower boiling points, the pores are larger and vice versa.11 Likewise, increasing the polymer concentrations from 1% to 5%, the porosity decreases about 25%.12 Other porogen agents have also been studied. For example, Qutachi et al prepared porous PLGA microspheres with an average size of 84 microns and pore size of 8 microns-15 microns by treatment for 2 mins with ethanolic sodium hydroxide (EtOH/NaOH) and used as injectable cell carriers.13
PREPARATION OF PLGA MICROSPHERES & DEVICES
Ruirui et al describe some of these methods used for delivery of a number of drugs encapsulated in PLGA micros/nano-spheres.14
Emulsion and double emulsion solvent evaporation process – (W/O/W) – this process is simple and requires the drug to dissolve in an aqueous solution (W) with or without excipients, and the PLGA is dissolved in organic solvents, preferably in methylene chloride or ethyl acetate (O). First W/O emulsions are formed by adding and mixing of aqueous solution of drug in PLGA dissolved in organic solvent. The first emulsions (W/O) are then mixed with a second continuous aqueous solution containing a surfactant, such as polyvinyl alcohol (PVA) to yield W/O/W emulsions. Following removal of organic solvent through diffusion or extraction or evaporation, it leads to solidification of the PLGA microspheres.
Phase separation or coacervation method – This requires the preparation of first emulsions by addition of aqueous drug into PLGA dissolved in methylene chloride. A coacervation agent, such as silicon oil, is gradually added into primary emulsions to promote phase separation. As methylene chloride is extracted into the silicon oil, PLGA microspheres get precipitated, which are further hardened by washing with apolar solvents, such as heptane or hexane.
Spray drying process – This atomization-based process requires the spray drying of W/O emulsions prepared by aqueous drug mixing with PLGA dissolved in methylene chloride. The resulting spray dried powder of PLGA microspheres entrapped with drug are dried to remove the residual solvents.
Hot melt extrusion (HME) – Hot melt extrusion is an ideal solvent-free method for creating implants by processing of PLGA at temperatures higher than glass transition temperature (Tg) of polymers, PLGA/PLA. The resulting extrudates or rods can be used as implants.
Nano-precipitation method – This process requires dissolving drug and PLGA in water-miscible organic solvents and injecting into an aqueous-phase water that leads to solvent exchange by diffusion and nanoprecipitation of drug into PLGA depots, ideally suited as reservoirs for in situ delivery.
Supercritical CO2 method – It involves the injection of drug dissolved in methanol into SC-CO2 as an anti-solvent, which acts as an extractant for methanol, and instantaneously lead to precipitation of drug nanoparticles, which is encapsulated in PLGA with well-dispersed drug in microspheres.
Electro-spraying process – It is based on atomization of drug and PLGA in an organic solvent, which is subjected to electric voltage to produce the particles. It is commonly used in preparation of solid dispersions of poorly soluble drugs.
Microfluidics technology – It offers good controls over particle size distribution and is amenable to low volumes. Microfluidic systems fitted with 1-mm channels have shown to be suitable for large-scale production of PLGA nanoparticles.
Membrane emulsification technology – It is relatively a new technology that employs a combination of emulsification methods and porous membranes in which the dispersed phase is pressed through a membrane, and the droplets formed are carried away with the continuous phase, which results in more uniform and controlled particle size with narrow distribution and high drug encapsulation efficiency than emulsification solvent evaporation technology.
PLGA NANOPARTICLES IN DRUG DELIVERY OF BLOOD BRAIN BARRIERS
The blood brain barrier (BBB) protects the central nervous systems (CNS) from unnecessary entry of unwanted substances, which also pose challenges in delivery of molecules for treatment of glioblastoma and other diseases in the brain. These tight junctions (TJs) formed by a complex network of proteins and linked with the cytoskeleton can restrict the passage of substances from the bloodstream to the brain, and as a result, many of the therapeutic agents can’t overcome the BBB. Kabanov et al have shown that poloxamer micelles (hydrophobic in nature) can penetrate the BBB and deliver the drugs.15 PLGA nanoparticles (hydrophobic in nature) can penetrate the BBB and can be used as carries for delivery of drugs.16 PLGA NPs with surface-modified surfactants also improved the cellular uptake by permeation of BBB. For example, PLGA NPs coated with vitamin E-TPGS, poloxamer 188, and polysorbate 80 improved the cellular uptake due, in part, to changes in surface hydrophilicity and surface charge.17 The MDCK cellular uptake of TPGS-coated PLGA (222 nm) was 1.5-fold higher compared to PVA-emulsified PLGA NPs following 4 hours of incubation. Likewise, with Poloxamer 188 and PS80-coated loperamide-encapsulated PLGA nanoparticles, the in vitro cellular uptake was 21% and 14.5%, respectively, compared to 4.5% uptake with unmodified PLGA nanoparticles with respect to free drug (ca. 0.4%). In vivo uptake differed considerably compared to in vitro cellular uptakes. Table 1 lists the brain uptake of surface-modified PLGA nanoparticles in animal models.16
It is evident from Table 1 that carotid administration showed relatively higher brain uptake than intravenous route of administration. For example, PS80-coated PLGA (231 nm) was delivered relatively higher than P188-coated PLGA nanoparticles (3.2%) at the same dose level by carotid route of administration in an hour. In other studies, P188 surface-modified PLGA nanoparticles were found to be better BBB targeting than PS 80 following intravenous administration.17
REGULATORY INFORMATION ABOUT PLGA & DRUGS APPROVED BY THE FDA
PLGA regulatory status is well established.18 It has been approved in many drug products (Table 2).19
PLGA is also listed in the FDA’s inactive ingredient database. Table 3 shows PLGA (free acid terminal) has been approved as implants and also in injectable solutions and suspensions for intravitreal, subcutaneous, and intramuscular drug products with maximum potency per unit and/or maximum daily exposure (MDE) limits. Evidently, the amounts of polymer could range from 135 mg to 533 mg per unit depending upon the formulation dosages to 11 mg-145 mg to MDE as injection suspension powders.20
ASCENDIA’S CAPABILITIES IN PLGA NANOPARTICLE FORMULATIONS
PLGA has been investigated in long-acting injectable formulations of a number of drug products from early phase to clinical phases of development. Ascendia’s in-house capabilities in upstream and downstream cGMP manufacturing of injectable lipid-based LipidSol® and polymeric-based PLGA have been well utilized within our state-of-the art sterile facility with ISO-certified cleanrooms. Table 4 shows the preparation of carvedilol- loaded PLGA nanoparticles from PLGA (50:50 LA/GA).21
The method for preparation of carvedilol-encapsulated PLGA nanoparticles involves dissolving the drug and polymer (1:10) in methylene chloride by thorough mixing as an oil phase. An aqueous solution containing 2% polyvinyl alcohol (PVA) with MW 9,000-10,000 D was used as a surfactant. API/polymer oil phase was added slowly in PVA aqueous solution dropwise and mixed thoroughly, keeping the oil/water phase ratio 1:7. The entire solution was mixed thoroughly in ice cold water to emulsify by sonication (Fisher Scientific Sonic Dismembrator Model 500) to achieve the desired particle size (Malvern Nano-ZS zeta sizer). The resulting nanoparticles were poured and mixed in 2% PVA aqueous solution for 3 hours, and collected following the centrifugation for 30 mins at 14,000 xg. The drug/PLGA nanoparticles were collected by discarding the supernatant and washed repeatedly with water. The resulting pure concentrated drug/PLGA nanoparticle suspension once again was passed through filter (Amicon® Ultra Centrifugal filter with MW 50 kD cut off), and centrifuged again for 10 mins at 14,000 xg to remove any free drug. It was lyophilized with sucrose (10%-30%) as cryo-protectant or used freshly or stored at 4°C for weeks, and drug loading was determined by HPLC, and release profile of drug was assessed.
SUMMARY
PLGA is widely used as an alternative drug delivery system for injectable drugs as a long-acting excipient. It forms microspheres that allows slow or control release of drugs. It is biocompatible and biodegradable, which makes it attractive for pharmaceutical drugs and medical devices. It is hydrophobic in nature with the ability to help hydrophobic drugs with limited ability for encapsulation of hydrophilic molecules. However, co-encapsulation with hydrophilic and hydrophobic drugs with high encapsulation efficiency is possible by changing the composition of the microsphere core-shell. Surface modification of PLGA with PEG or receptors can lead to longer circulation and targeting of certain disease tissues, respectively. PLGA microspheres can increase drug loading and avoid phagocytosis by macrophages, thus achieving a longer lasting drug-release effect. Because of their smaller particle size and targeting characteristics, they are easier to accumulate in tumor cells through the enhanced permeability and retention (EPR) effect. In addition to therapeutic molecules, imaging agents can also be encapsulated in w1/o/w2 double emulsions to help improve cancer cells targeting and bioavailability of hydrophobic molecules. Choice of selecting non-toxic solvents also adds another significant step forward to select PLGA for designing a safe vehicle for encapsulating and delivering drugs across all modalities. Designing PLGA micro/nanospheres requires smarter selection of the fabricating process. For example, membrane emulsion and microfluidics methods yield PLGA microspheres with higher drug encapsulation efficiency and with lower polydispersity index, for example, PDI of 0.048 with carvedilol encapsulated PLGA.21 Taken collectively, Ascendia’s capabilities in the downstream and upstream manufacturing process can lead to development of PLGA-based nanoparticle-encapsulated drugs for life-threatening ailments. State-of-the-art cGMP manufacturing suites designed to handle organic solvents, including methylene chloride, are well in place for future generations of polymeric and lipid-based nanoparticles for unmet medical needs.
REFERENCES
- P. Blasi, Poly(lactic acid)/poly(lactic-co-glycolic acid)-based microparticles: An overview. J. Pharm. Invest. 2019, 49, 337–346.
- X. Shen, T. Li, X. Xie, Z. Chen, H. Yang, C. Wu, S. Deng and Y. Liu, PLGA based drug delivery systems for remotely triggered cancer therapeutic and diagnostic applications, Frontiers Bioeng. Biotech., 2020, 8, 381. doi:10.3389/fbioe.2020.00381.
- M. Alvi, A. Yaqoob, K. Rahman, S. M. Shoaib and M. S. H. Akash, PLGA based nanoparticles for the treatment of cancers: current strategies and perspectives, AAPS Open, 2022, 8, 12;https://doi.org/10.1186/s41120-022-00060-7.
- P. Gentile, V. Chiono, I. Carmagnola, and P. V. Hatton, An overview if poly(lactide-co-glycolic) acid (PLGA) -based biomaterials for bone tissue engineering, Int. J. Mol. Sci., 2014, 15,3640-3659.
- E. Lagreca, V. Onesto, C. D. Natale1, S. L. Manna, P. A. Netti and R. Vecchione, Recent advances in the formulation of PLGA microparticles for controlled drug delivery, Prog. Biomaterials, 2020, 9, 153-174.
- H. K. Makadia and S. J. Siegel, Poly Lactic-co-Glycolic Acid (PLGA) as Biodegradable Controlled Drug Delivery Carrier, Polymers, Polymers, 2011, 3, 1377–1397. doi:10.3390/polym3031377.
- M. Dunne, I. Corrigan, and Z. Ramtoola, Influence of particle size and dissolution conditions on the degradation properties of polylactide-co-glycolide particles. Biomaterials. 2000; 21:1659 -1668.
- Z. Yu, L. Huang, R. Wen, Y. Li and Q. Zhang, Preparation and in vivo pharmacokinetics of rhGH-loaded PLGA microspheres Pharm. Dev. Technol., 2019, 24 395–401.
- J. Lee, Y. J. Oh, S. K. Lee, and K. Y. Lee, Facile control of porous structures of polymer microspheres using an osmotic age pulmonary delivery. J Control Release, 2010, 146, 61–67.
- C. Busatto, J. Pesoa, I. Helbling, J. Luna, and D. Estenoz (2018) Effect of particle size, polydispersity and polymer degradation on progesterone release from PLGA microparticles: experiment and mathematical modeling. Int. J. Pharm. 2018, 536, 360–369.
- J. Wang, L. Helder, J. Shao, J. A. Jansen, M. Yang and F. Yang, (2019) Encapsulation and release of doxycycline from electrospray-generated PLGA microspheres: effect of polymer end groups. Int J Pharm., 2019, 564,1–9.
- B. Amoyav, and O. Benny, Microfluidic based fabrication and characterization of highly porous polymeric microspheres. Polymers, 2019, 11, 419.
- O. Qutachi, O., R. Jolanda, D. G. Vetsch, H. Cox, H., D. J. Scurr, S. H. R. Müller, R. A. Quirk, K. M. Shakesheff, and C. V. Rahman, Injectable and porous PLGA microspheres that form highly porous scaffolds at body temperature, Acta Biomater., 2014 10, 5090–5098.
- Z. Ruirui, J. He, X. Xu, S. Li, H. Peng, Z. Deng and Y. Huang, PLGA-based drug delivery system for combined therapy of cancer: research progress, Mater. Res. Express, 2021, 8, 122002.
- A. Kabanov, J. Zhu and V. Alakhov, Pluronic Block Copolymers for Gene Delivery. Advances in Genetics. 2005, 53, 231-261. DOI: 10.1016/S0065-2660(05)53009-8.
- J. Li and C. Sabliov, PLA/PLGA nanoparticles for delivery of drugs across the blood-brain barrier, Nanotechnol Rev. 2013, 2, 241–257; DOI 10.1515/ntrev-2012-0084.
- S. Gelperina, O. Maksimenko, A. Khalansky, L. Vanchugova, E. Shipulo, K. Abbasova, R. Berdiev and S. Wohlfart, Drug delivery to the brain using surfactant-coated poly(lactide-co-glycolide) nanoparticles: Influence of the formulation parameters. Eur J Pharm Biopharm. 2010, 74, 157-163.
- Y. Wang, B. Qin, G. Xia and S. H. Choi, FDA’s poly (lactic-co-glycolic acid) research program and regulatory outcomes, AAPS J., 2021, 23, 92.
- A. Gonella, S. Grizot, F. Liu, A. L. Noriega and J., Richard, Long-acting injectable formulation technologies: challenges and opportunities for the delivery of fragile molecules, Expert Opinion Drug Deliv., 2022, 19, 927–944.
- FDA Inactive ingredient database for PLGA,https://www.accessdata.fda.gov/scripts/cder/ iig/index.cfm?event=BasicSearch.page.
- V. Ivanova, K. Maeda, W. Wang, D. Guo and J. Huang, Parenteral sustained release delivery of carvedilol disperse systems, US Patent, 10,792,244 (October 6, 2020).
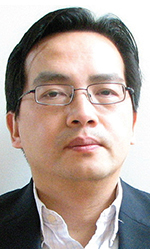
Jim Huang, PhD
Founder & CEO
Ascendia Pharmaceuticals
j.huang@ascendiapharma.com
www.ascendiapharma.com
Dr. Jim Huang is the Founder and CEO of Ascendia Pharmaceuticals, Inc. he earned his PhD in Pharmaceutics from the University of the Sciences in Philadelphia (formerly Philadelphia College of Pharmacy and Sciences) under Joseph B. Schwartz. He has more than 20 years of pharmaceutical experience in preclinical and clinical formulation development, manufacturing, and commercialization of oral and parenteral dosage forms. His research interests are centered on solubility/bioavailability improvement and controlled delivery of poorly water-soluble drugs through nano-based technologies.
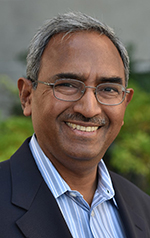
Shauket Ali, PhD
Sr. Director, Scientific Affairs & Technical Marketing
Ascendia Pharmaceuticals
shaukat.ali@ascendiapharma.com
www.ascendiapharma.com
Dr. Shaukat Ali joins Ascendia Pharmaceuticals Inc. as Senior Director of Scientific Affairs and Technical Marketing after having worked in the pharma industry for many years. His areas of expertise include lipid chemistry, liposomes, lipid nanoparticles, surfactant-based drug delivery systems, SEDDS/SMEDDS, oral and parenteral, topical and transdermal drug delivery, immediate- and controlled-release formulations. He earned his PhD in Organic Chemistry from the City University of New York and carried out his post-doctoral research in Physical Biochemistry at the University of Minnesota and Cornell University. He has published extensively in scientific journals and is inventor/co-inventor of several US and European patents.
Total Page Views: 8435