Issue:January/February 2024
FORMULATION FORUM - Lipid Nanoparticles – Carriers for Nucleic Acids Delivery
INTRODUCTION
Nucleic acid therapeutics has emerged as a new class of potential drugs for targeting and treatment of various diseases. In recent years, there has been a considerable interest in finding the appropriate technologies to formulate nucleic acids into an appropriate and bioavailable dosage form, especially mRNA.1 This continued interest in nucleic acid encapsulation for vaccines stems from their ability to induce antigen expression and adaptive immune responses from encoded antigen safely and effectively. As a result of the introduction of life-saving Covid 19 vaccines for severe acute respiratory syndrome coronavirus (SARS-CoV-2), Spikevax (Moderna) and Comirnaty (Pfizer/BioNTech), and the launch of small interfering RNA (siRNA) as a polyneuropathy drug by Alnylam, the industry is renewing interest in the next generation of lipid nanoparticle technology for the delivery of mRNA therapeutics for cancers, anti-inflammatories, and rare diseases, among others.2 With modern synthetic technologies, mRNA can be produced at a large scale by an enzymatic manufacturing process, making nucleic acids more affordable for larger populations with unmet medical needs.2 As shown in Figure 1, the innovation in LNP technologies has made it possible for the commercial launch of several small molecules, peptides, nucleic acids, and biologics to the market.3
Due to their complex structure, encapsulation and delivery of nucleic acids by LNPs in an efficient and effective manner creates challenges and opportunities for the pharma industry. For instance, these lipid assemblies have been proven to be ideal vehicles for intracellular delivery of mRNA due to their inherent abilities to protect the delicate nucleic acids from degradation under aqueous and nuclease environment.4-7 However, the encapsulation of mRNA into LNPs warrants a closer scrutiny because every component of LNPs, especially cationic lipids, plays a crucial role in protecting, delivering, and stabilizing these large macromolecules.8
The following describes the role of individual components in aggregation, packing, stability, efficacy, and potency of nucleic acids, the understanding of which is important to achieve better designed and smarter formulations, and robust scale up and manufacturing of LNPs.
STRUCTURE OF LNPS WITH NUCLEIC ACIDS
To design a robust lipid nanoparticle, a comprehensive understanding of lipid components is required. LNPs used in encapsulation of negatively charged nucleic acids are typically composed of four components, all of which are synthetic in nature as shown in Figure 2.
The formulation compositions of mRNA LNPs are composed of four lipids.9 For example, the SPIKEVAX® formulation by Moderna for each 0.5-mL dose contains: 50 mcg of nucleoside modified messenger RNA: mRNA and total lipid content of 1.01 mg (SM-102, polyethylene glycol [PEG] 2000 dimyristoyl glycerol [DMG], cholesterol, and 1,2-distearoyl-sn-glycero-3- phosphocholine [DSPC]).
While, COMIRNATY® by Pfizer for each 0.3-mL dose contains: lipids (0.43 mg [(4-hydroxybutyl)azanediyl) bis(hexane-6,1-diyl)bis (2-hexyldecanoate), 0.05 mg (polyethylene glycol 2000)-N,N-ditetradecylacetamide, 0.09 mg 1,2-distearoyl-sn-glycero-3-phosphocholine, and 0.19 mg cholesterol].
For ONPATTRO® (Patisiran), each 1-mL dose contains 6.2 mg cholesterol USP, 13.0 mg [6Z,9Z,28Z,31Z)-heptatriaconta6,9,28,31-tetraen-19-yl-4-(dimethylamino) butanoate (DLin-MC3-DMA), 3.3 mg 1,2- distearoyl-sn-glycero-3-phosphocholine (DSPC), 1.6 mg α-(3’-{[1,2- 2 di(myristyloxy)propanoxy] carbonylamino}propyl)-ω-methoxy, polyoxyethylene (PEG -C-DMG)]. The details of formulation compositions of these products are outlined in Table 1 and are published elsewhere.10
Most of the ingredients used in the marketed drugs are also listed in the FDA inactive ingredient database. However, how these lipids are distributed within the complex nanostructured LNPs has been debated.11,12 It is obvious the negatively charged nucleic acid is complexed with positively charged ionizable lipids (as a lipoplex) and is entrapped within the LNPs. For LNPs designed with helper phospholipids, such as di-stearoyl phosphocholine and cholesterol, these lipids are distributed asymmetrically to create an outer stable monolayer boundary, whereas the pegylated lipids are situated outside for providing steric stability of the core surface. Efforts are still being made by researchers to design better and smarter ionizable lipids to yield greater nucleic acid stability and fusogenicity.13,14 Likewise, several of the PEGylated lipids are discovered aiming at sterically stabilized LNPs with longer circulation time in the blood without being opsonized. Unlike larger mRNA, it is believed siRNA nucleic acid is encapsulated within a spherical multilamellar structure in which the nucleic acid is sandwiched between bilayer assemblies.15 Cryo-EM and small angle X-ray scattering could shed some light on the packing of nucleic acids within the LNPs; however, because the mass density contrast is not appreciably distinctive enough to resolve RNA from the lipid components, attempts are still continuously made to identify the RNA within the lipid nanoparticles. Brader, et al (2021) used a cationic dye (thionine) as a contrast agent in cryogenic electron microscopy (Cryo-EM); they found the chemical microenvironment of mRNA appears to be located within solvent-filled cavities and fully associated with lipids (Figure 3).16 According to a recent study, it appears the LNP interior is composed of electrostatically neutral inverted micelles in which nucleic acid is surrounded by the ionizable cationic lipid and other lipid components, whereas the surface of the LNP is composed of a hydrophilic shell containing the PEG-lipid.17
McKenzie et al (2023) used ionizable lipids, for example, Dlin-MC3-DMA (MC3), to design mRNA-encapsulated LNPs.18 In this method, the authors used a microfluidic mixer to control the nanoprecipitation of lipid and mRNA in acidic buffer followed by the pH adjustment step with phosphate buffer that yielded stable LNPs with 80-250-nm diameters in size and encapsulation efficiency of > 80%. Different ionizable lipids have been used to design LNPs for encapsulation of mRNA.14 Jayaraman, et al (2012) prepared stable LNPs from a composition composed of ionizable lipid/DSCP/Cholesterol/DMG-PEG-2000 (50:10:38.5:1.5), with an N/P ratio of 4 (N being the ionizable amine and P being the phosphate associated with mRNA).19 The PEGylated lipids and helper phospholipids are in the range of 1%-2% and 8%-12%, respectively. The typical Helper phospholipid used is DSPC (1,2-distearoyl-sn-glycero-3-phosphocholine) that can help to yield highly stable LNPs. DOPE (dioleoyl-sn-glyecro-3-phosphatidyl ethanolamine) is also used to form a cone fluidic structure as opposed to a cylindrical stable structure of DSPC. The percentage of ionizable lipid could range from 40%-50% to create stable LNPs and promote fusogenicity for transfection efficacy. There are a few case studies to identify novel ionizable lipids to stabilize mRNA-encapsulated LNPs at subzero and nonfrozen conditions.20 COVID-19 mRNA vaccines are stored in freezing conditions with added sucrose to provide additional stability. Other studies tried to optimize the mRNA nucleotide composition to help stabilize the LNPs by using lyophilization technology.21
MECHANISM OF RNA RELEASE & IMMUNE RESPONSE
Ionizable lipid nanoparticles (iLNPs) are composed of cationic lipids with amino moiety as the head group, PEGylated lipid, and the helper lipids, including phospholipid and cholesterol, which provide the stability of the outer layer core. The amino head group of the ionizable lipids are typically tertiary amine with a pKa of 6.2-6.9. The ionizable lipids available commercially are MC3 and ALC-0315 in the Comirnaty vaccine (Pfizer/BioNTech), and SM-102 in the Spikevax vaccine (Moderna). All these lipids are protonated at an acidic pH but are neutral at physiological pH. Their structures are such that they help LNPs to fuse with endosomal membranes while being recognized at the cell surface due to their positive charge. As shown in Figure 4, once LNPs are endocytosed into the cell, they release mRNA into cytosol via endosomal scape, which instructs the cell to produce spike proteins that in turn triggers the immune response and produces the antibodies to fight the viruses.3
MANUFACTURING LNPS
Because these amphiphilic lipids can spontaneously aggregate into LNPs in aqueous solution, the process to make LNPs only requires direct mixing of lipid (organic) and nucleic acids (aqueous solution) by agitation that results in encapsulation of negatively charged mRNA. Hydrophobic fatty acid chains and polar headgroups help create the lipid assemblies that can further be sized into desired particle sizes. Top down and bottom-up approaches are commonly used for generating these particles. Top-down approach requires high shear and high energy in which the lipid (dried film) is hydrated and homogenized in aqueous buffer to yield a desired particle size. The bottom-up approach, such as nanoprecipitation, requires the ethanol injection that results in the formation of nanoparticles, but this method suffers from uncontrolled particle size due to inhomogeneous mixing.22 To alleviate such challenges, microfluidic mixing is highly sought after for generating desired particle sizes with uniform size distribution under the laminar flow conditions. This technique is fast and easy to scale up for manufacturing of large batches of LNPs. Precise control of mixing through T-junction or staggard herringbone mixer or ring mixer prevents premature premixing and results in uniform particle size distribution with low polydispersity under precisely controlled temperature and flow rate.23 Shepherd, et al (2023) developed a microfluid chip method (so-called SCALAR) for producing the LNPs with throughput of >17 L /h at commercial manufacturing scale compared to >10 L/h device available commercially by Precision Nanoassemblr.24 Based on silicon and glass substrates, these chips (each 100 mm in length) are solvent compatible, stable at higher temperature (>500°C) and pressure (100 pounds per square). Designed and fabricated with arrays of 256 parallel mixing units, this microfluidic device yields precisely well-defined potent and robust mRNA-LNPs. Using SCALAR 256x chip and PolyA as substrate (a mRNA substitute), Shepherd, et al demonstrated the formation of LNPs (composed of ionic lipid D-Lin-MC3-DMA:DSPC:Cholesterol:DMG-PEG 2000; 50:10:38.5:1.5) in high throughout production with uniform particle size (ca. 70 nm by intensity-weighted average), low polydispersity index (PDI), high encapsulation efficiency, and comparable in vivo data in mice.24
Hengelbrock, et al (2023) used the continuous manufacturing process for LNPs encapsulated with mRNA through microfluidic mixing (using a T-mixer) that meet the specifications and critical quality attributes of the products with a high encapsulation efficiency (ca. 88% EE),consistent particle size (ca. 71 nm), and low polydispersity index of 0.004.25 These attributes are aligned with the target profile (ca. 66-93 nm and 88% EE) of Pfizer’s Comirnaty mRNA vaccine. The scale up manufacturing requires a T- or Y-mixer that allows for rapid mixing of lamellar flow liquids into a turbulent flow at the mixing point with a Reynolds number of 11,000.26 By changing the lipid composition and the flow rate ratio between the two phases (organic/aqueous), the size of LNPs can be controlled. For typical mRNA encapsulation, one part of lipid solution is mixed with three parts of aqueous phase. Downstream tangential flow filtration (TFF) is used to concentrate the LNPs to remove ethanol, any residual lipids and non-encapsulated nucleic acids, and to neutralize the formulation to the target pH of 7.4. The authors demonstrated that for LNPs generated by the continuous T- or Y- mixing modes, those formulations meet the quality critical attributes of mRNA vaccines with particle size of 71 nm and EE of 88%. This continuous process can significantly save time and costs.
Other manufacturing devices applied for large-scale production of LNPs include the impingement jet mixer (IJM), which involves mixing of two fluids at a high-velocity stream, resulting in effectively homogenization at intense shearing forces. This process has now been used in manufacturing COVID-19 vaccines. For instance, Knauer utilizes IJM system, which allows the mixing of lipid solvent solutions with an mRNA aqueous solution at 400 pounds pressure with a controlled flow to effectively force the two fluids to mix. Pfizer has also used this method successfully to significantly increase the vaccine productivity to 100 million doses per month.27 Thus, IJM is a unique manufacturing process for producing uniform, robust, and stable LNPs for drug delivery applications. Maeki et al (2023) used a microfluidic design comprising five layered microchannels created in parallel stacking glass-iLiNP (invasive lipid nanoparticle) devices to achieve mass production.28 This iLiNP device efficiently produces lipid nanoparticles with 20 to 60 nm sizes at a flow rate of 20-50 ml/min in a continuous mode as the NanoAssemblr’s microfluidic commercial device.
LipidSol® by Ascendia Pharma is an LNP platform technology that provides different process methods, such as microfluidics, thin film hydrating, extrusion, high pression homogenization, nanoprecipitation, and emulsification/double emulsification to make LNPs for various therapeutic modalities with both hydrophilic and lipophilic properties.4 Coupled with lab-scale screening and cGMP sterile manufacturing capabilities, Ascendia is leading the way in design, development, and manufacturing of LNPs for novel therapeutics for treatment of cancers and infectious and many other rare diseases.
SUMMARY
LNPs offer an innovative drug delivery method for targeting certain tissues with mRNA lipoplexes composed of ionizable lipids varied in their structures and properties. This will open the doors for numerous opportunities in drug delivery of biologics due to efficient encapsulation and greater protection of the nucleic acid within LNP cargo.29 As a result of such technologies, many new and innovative drugs are undergoing clinical studies for delivery of mRNA for cancer vaccines and immunogenic therapeutics.30 For example, mRNA-4150 and mRNA-5671 vaccines are undergoing the clinical phases for treatment of specific melanoma cancer and non-small cell lung cancer, respectively.31 Likewise, there are many other clinical studies with mRNA ongoing for development of several therapeutics in LNPs for cancer vaccines and for treatment of infectious diseases.3 As we continue to search for targets, it is our understanding that LNP-mRNA technologies hold a greater promise for future drug development in finding the cures of life-threatening diseases.
Supporting the development of mRNA technologies for innovative medicines for unmet medical needs, CDMOs with the right expertise and in-house capabilities will play a key role in providing the greater flexibility in manufacturing of modern medicines.32 More specifically, CDMOs offering a one- stop solution with end-to-end services from formulation, process development to cGMP manufacturing with fill-finish capabilities will accelerate the drug development process to market.
REFERENCES
- Nature Editorial. Let’s talk about lipid nanoparticles, Nature Reviews – Materials. 2021;6:99.
- N. Chaudhary, D. Weissman and K.A. Whitehead. mRNA vaccines for infectious diseases: principles, delivery and clinical translation, Nat. Rev. Drug Disco. 2021:20:817-838.
- R. Tenchov, R. Bird, A.E. Curtze, et al. Lipid nanoparticles from liposomes to mRNA vaccine delivery, a landscape of research diversity and advancement. ACS Nano. 2021;15:16982-17015.
- J. Huang, S. Ali. LIPIDSOL®: Liposomes – Chemistry, Properties & Applications of Lipid Nanoparticles. Drug Development & Delivery. 2023. https://drug-dev.com/formulation-forum-lipidsol-liposomes-chemistry-properties-applications-of-lipid-nanoparticles/.
- S. Guan and J. Rosenecker. Nanotechnologies in delivery of mRNA therapeutics using nonviral vector-based delivery systems. Gene Ther. 2017;24:133-143.
- A.M. Reichmuth, M.A. Oberli, A. Jaklenec, et al. mRNA vaccine delivery using lipid nanoparticles. Therapeutic Delivery. 2016:7:319-334.
- S.C Semple, A. Akinc, J. Chen, et al. Rational design of cationic lipids for siRNA delivery. Nature Biotech. 2010;28:172-176.
- C. Gueguen, T. Ben Chimol, M. Briand, et al. Evaluating how cationic lipid affects mRNA-LNP physical properties and biodistribution. Eur. J. Pharm. Pharmacol. DOI: https://doi.org/10.1016/j.ejpb.2023.08.002.
- NIH: Daily Med (https://dailymed.nlm.nih.gov/dailymed/).
- Y. Suzuki and H. Ishihara. Difference in the lipid nanoparticle technology employed in three approved siRNA and mRNA (Covid-19 vaccine) drugs. Drug Metab. Pharmaco. 2021;41:100424.
- A.K.K. Leung, Y.C. Leung, S.C. Tam, et al. Microfluidic mixing: A general method for encapsulating macromolecules in lipid nanoparticle systems. J. Phys. Chem. B. 2015;119:8698-8706.
- J.A. Kulkarni, M.M. Darjuan, J.E. Mercer, et al. On the formation and morphology of lipid nanoparticles containing ionizable cationic lipids and siRNA. ACS Nano. 2018;22;12(5):4787-4795. doi: 10.1021/acsnano.8b01516. Epub 2018 Apr 6.
- X. Hou, T. Zaks, R. Langer, et al. Lipid Nanoparticles for mRNA delivery. Nature Reviews. 2021;6:1078-1094.
- F. DeRosa, S. Karve, and M. Heartlein, Encapsulation of messenger RNA. US Patent No. 9,668,980 (June 6, 2017).
- M. Gindy, K. DeFelice, and Boardman. Mechanisms of macromolecular structure evolution in self-assembled lipid nanoparticles for siRNA delivery. Langmuir. 2014;30:4613-4622.
- M.L. Brader, S.J. Willims, J.M. Banks, et al. Encapsulation state of messenger RNA inside lipid nanoparticles. Biophys. J. 2021;120:2766-2770.
- R.N. Kularatne, R.M. Crist, and S.T. Stern. The future of tissue-targeted lipid nanoparticle-mediated nucleic acid delivery. Pharmaceutics. 2022;15: 897;doi.org/10.3390/ph15070897.
- R.E. McKenzie, J.J. Minnell, M. Ganley, et al. mRNA synthesis and encapsulation in ionizable lipid nanoparticles. Current Protocols. 2023;3:1-47.
- M. Jayaraman, S.M. Ansell, B.L. Mui, et al. Maximizing the potency of siRNA lipid nanoparticles for hepatic gene silencing in vivo. Angew. Chemie Inter. Ed. 2012;51(34):8529-8533. https://doi.org/10.1002/anie.201203263.
- A.G. Reinhart, A. Osterwald, P. Ringler, et al. Investigations into mRNA lipid nanoparticles shelf-life stability under nonfrozen conditions. Mol. Pharm. 2023 (in press); doi.org/10.1021/acs.molpharmaceut.3c00956.
- L. Schoenmaker, D. Witzigmann, J.A. Kulkarni, et al. mRNA-lipid nanoparticle Covid-19 vaccines: structure and stability. Int. J. Pharm. 2021;601:120586.
- M. Mehta, T.A. Bui, X. Yang, et al. Lipid-based nanoparticles for drug/gene delivery: an overview of the production techniques and difficulties encountered in their industrial development. ACS Mater. 2023;3:600-619.
- Ripolle M, Martin E, Enot M, Robbe O, et al. Optimal self-assembly of lipid nanoparticles (LNP) in a ring micromixer. Scientific Reports. 2022;12(1): 9483.
- S.J. Shepherd, X. Han, A.J. Mukalel, et al. PNAS. 2023;120:1-12.
- A. Hengelbrock, A. Schmidt, and J. Stube. Formulation of nucleic acids encapsulation in lipid nanoparticles for continuous production of mRNA. Process. 2023;11:1718.
- S. Hirota, C.T. De Ilarduya, L.G. Barron, et al. Simple mixing device to reproducibly prepare cationic lipid-DNA complexes (lipoplexes). Biotechniques. 1999;27:286-290.
- N. Warne, M. Ruesch, P. Siwik, P, et al. Delivering 3 billion doses of Comirnaty in 2021. Nat. Biotechnol. 2023;41:183-188.
- M. Maeki, Y. Okada, S. Uno, et al. Mass production system for RNA-loaded lipid nanoparticles using piling up microfluidic devices. Applied Materials Today. 2023;31:101754.
- M.L. Guevara, F. Persano, and S. Persano. Advances in lipid nanoparticles for mRNA-based cancer immunotherapy. Front. Chem. 2020;8:589959.
- L. Miao, Y. Zhang, and L. Huang. mRNA vaccine for cancer immunotherapy. Mol. Cancer. 2021;20:41.
- Moderna Inc. Available online: https://www.modernatx.com/research/product-pipeline (accessed on 3 June 2022).
- E. Park. Meeting manufacturing demand in the new era of mRNA technologies. Contract Pharma. 2023;12:18-20.
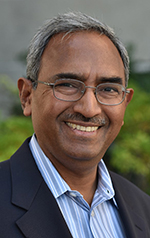
Shauket Ali, PhD
Sr. Director, Scientific Affairs & Technical Marketing
Ascendia Pharmaceuticals
shaukat.ali@ascendiapharma.com
www.ascendiapharma.com
Dr. Shaukat Ali joins Ascendia Pharmaceuticals Inc. as Senior Director of Scientific Affairs and Technical Marketing after having worked in the pharma industry for many years. His areas of expertise include lipid chemistry, liposomes, lipid nanoparticles, surfactant-based drug delivery systems, SEDDS/SMEDDS, oral and parenteral, topical and transdermal drug delivery, immediate- and controlled-release formulations. He earned his PhD in Organic Chemistry from the City University of New York and carried out his post-doctoral research in Physical Biochemistry at the University of Minnesota and Cornell University. He has published extensively in scientific journals and is inventor/co-inventor of several US and European patents.
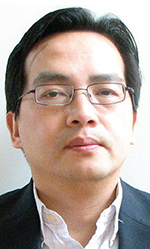
Jim Huang, PhD
Founder & CEO
Ascendia Pharmaceuticals
j.huang@ascendiapharma.com
www.ascendiapharma.com
Dr. Jim Huang is the Founder and CEO of Ascendia Pharmaceuticals, Inc. he earned his PhD in Pharmaceutics from the University of the Sciences in Philadelphia (formerly Philadelphia College of Pharmacy and Sciences) under Joseph B. Schwartz. He has more than 20 years of pharmaceutical experience in preclinical and clinical formulation development, manufacturing, and commercialization of oral and parenteral dosage forms. His research interests are centered on solubility/bioavailability improvement and controlled delivery of poorly water-soluble drugs through nano-based technologies.
Total Page Views: 6790