Issue:October 2022
EXTRACELLULAR VESICLES - Engineering Extracellular Vesicles to Create Next-Generation Therapeutics
INTRODUCTION
Cells from both eukaryotes and bacteria release extracellular vesicles (EVs) as a form of intercellular communication and as a method to regulate biological processes.1,2 EVs can be broadly defined based on their size and method of cellular biogenesis. Exosomes are small (approximately 30 nm to 150 nm in diameter) extracellular membranous vesicles formed through invagination of the endosomal membrane to form an endosomal multivesicular body (MVB), which fuses with the plasma membrane, releasing the intraluminal vesicles as exosomes.3 This contrasts with other extracellular vesicles, such as microvesicles (which can range from approximately 100 nm to >1000 nm in diameter), which are released through outward budding of the plasma membrane, or apoptotic bodies, produced during apoptotic cell death.4 EVs have been shown to be capable of naturally transporting a wide variety of cellular metabolic cargoes, such as proteins, lipids, transcription factors, miRNAs, and mRNA, and it is this natural role of exosomes as “nature’s delivery vehicle” that has led to much interest in their potential exploitation for therapeutic use. Most of this therapeutic interest is focussed on exosomes, but as there is still much work to be done in characterising the different classes of EVs from both a biological function, as well as a transcriptomic and proteomic perspective, this review will refer to all types of these bodies as EVs, rather than differentiating between exosomes, microvesicles, or apoptotic bodies, and will focus on emerging engineering approaches to harness the therapeutic potential of EVs.1
NON-ENGINEERED EXOSOME THERAPIES
There is now a vast and rapidly growing body of data demonstrating the role of EVs in both physiological and pathological processes.2,5 EVs released by tumor cells have been associated with increased tumor invasiveness and metastasis.6,7 EVs have also been shown to contribute to the pathology of atherosclerotic plaques, as well as potentially playing a role in the spread of misfolded neurotoxic proteins, such as prion proteins, amyloid beta, and alpha-synuclein, associated with the pathology of neurodegenerative diseases.8 In addition, the multiple physiological roles EVs play in healthy cell-to-cell communication has led to much recent interest in their therapeutic application. In vivo studies using EVs derived from multiple cell sources have demonstrated therapeutic potential in models of varied diseases, such as cancer, stroke, myocardial ischaemia, as well as vaccines to protect from infections from bacteria and other pathogens. More excitingly, in recent years, human clinical studies have been initiated with EVs derived from mesenchymal stem cells (MSCs) in several therapeutics areas, such as graft-versus-host disease and chronic kidney disease. ExoPharm (Melbourne, Australia) have conducted a successful clinical trial with platelet-derived EVs in healthy volunteers undergoing an experimental skin punch biopsy-induced wound (PLEXOVAL II). The potential use of dendritic cell-derived EVs as vaccines is also under clinical investigation, making use of the presence on these EVs of immunostimulatory proteins and MHC-peptide complexes. The clinical progression of these platelet, MSC, and other cell-derived EV products will necessitate the development of scaled-up manufacturing procedures, particularly in terms of purification and characterisation methods, as well as analytical techniques to determine product quality and reproducibility. Similarly, the novel nature of EVs as a therapeutic modality will necessitate establishing regulatory paths to the clinic and eventually the marketplace. In order to unlock the full potential of EVs as a therapeutic modality, multiple laboratories are investigating approaches to engineer EVs, through genetic manipulation of EV-associated proteins in the parental cell-line to introduce additional adventitious properties, such as cell or tissue targeting or directed loading of a therapeutic cargo. Additionally, there is much interest in treating EVs as a drug delivery nanoparticle for the loading and delivery of therapeutic molecules, such as chemotherapies and small interfering RNA (siRNA). These exciting approaches will form the bulk of this review.
ENGINEERED EVS AS POTENTIAL THERAPEUTICS
Releasing the inherent potential of EVs as therapeutics and as delivery vehicles for therapeutic cargoes will likely require the optimization of multiple EV characteristics. Depending on the clinical indication and suggested route of administration, some of these might include altering the biodistribution and pharmacokinetics of EVs, as well as maximizing the loading of the therapeutic cargo, either on the surface, or within the lumen of the EV. In order to try to engineer these properties, much research has focussed on identifying and understanding the roles of EV-enriched and associated molecules.9,10
EV-ASSOCIATED PROTEINS AS ENGINEERING SCAFFOLDS
The discovery and characterization of the different types of EV rapidly led to proteomic and mechanistic analyses to identify proteins associated with their formation, endogenous cargo loading, secretion, targeting, and uptake.9 Several key protein families that were found to be enriched in EVs including the tetraspanins, particularly CD9, CD63, and CD81 and members of the ESCRT machinery, such as Alix and TSG-101. Other proteins enriched on or within EVs include lysosome-associated membrane proteins (LAMPs), heat shock proteins (HSPs), major histocompatibility complex (MHC) proteins, adhesion molecules such as intercellular adhesion molecules (ICAMs), integrins and selectins, as well as a wide range of proteins associated with membrane transport and fusion, such as annexins, and GTPases.11 More recently, the EWI immunoglobulin superfamily member PTGFRN and the MARCKS protein family member BASP1 have been reported to be highly enriched in EVs and to show great versatility as engineering scaffolds.12 Several of these protein classes, in particular the tetraspanins, and the LAMPs have been successfully used as EV-associated scaffolds for engineering across a wide range of biological contexts (Figure 1).
ENGINEERING EVS FOR SPECIFIC TROPISM
EVs typically have rapid pharmacokinetics, with serum half-lives of a few minutes in both rodent and non-human primate (NHP) studies.13 These studies have shown that systemically delivered EVs rapidly accumulate in the liver and spleen. In order to widen the therapeutic potential of EVs extrahepatically, exosomal scaffold proteins have been engineered with tissue-targeting moieties. An early example of such an approach was the insertion of a peptide derived from the rabies virus glycoprotein (RVG), which has been shown to facilitate drug delivery into the central nervous system into the EV surface associated protein LAMP2B.14,15 In this study, RVG-LAMP2B-expressing EVs could be shown to functionally deliver siRNA cargo to the CNS, following intravenous injection in mice. Other strategies for optimizing EV transit across the blood-brain barrier include conjugating EVs with anti-CD22.16 Engineering EVs to display antibodies or antibody fragments is an increasingly powerful way to direct the tropism of the EV to a particular target tissue or cell. Shi et al engineered both anti-CD3 and anti-HER2 single-chain Fv (scFv) fragments fused to the human platelet-derived growth factor receptor (PDGFR) transmembrane domain to generate exosomes that could simultaneously target T cell CD3 and breast cancer expressed HER2 and which exhibited potent in vitro and in vivo activity.17 Similarly, Dooley et al successfully fused anti-CD3 antibody fragments to the PTGFRN scaffold protein, resulting in functional engagement with murine T cells.12
ENGINEERING EVS FOR ENHANCED PHARMACOKINETICS
As previously noted, systemically administered EVs are rapidly cleared from the serum and accumulate to a significant extent in the liver and spleen within minutes of dosing. While this type of profile can be desirable in certain clinical settings, engineering approaches that can a) extend the serum half-life in order to facilitate a wider biodistribution, enabling delivery to therapeutic sites of interest, and/or b) prevent EV phagocytosis by macrophages, will likely be required for a successful EV therapeutic in many clinical scenarios. A well-validated approach to extending serum half-life for therapeutic molecules is by binding to serum albumin.18 Our laboratory has recently incorporated an albumin-binding domain peptide into the extracellular loop of the tetraspanin CD63 and have shown that this extends the serum persistence of EVs following systemic dosing in mice (manuscript in preparation).
Another approach using CD47 involves preventing EV phagocytosis by macrophages. CD47 is an integrin associated transmembrane protein that is the ligand for signal regulatory protein alpha (SIRPα). CD47-SIRPα binding initiates the “don’t eat me” signal that inhibits phagocytosis and was shown by Kalluri and colleagues to help EVs avoid rapid degradation by professional phagocytes.19 Overexpression of such molecules in EVs may represent a strategy to camouflage therapeutic EVs to avoid phagocytosis and thus enhance their pharmacokinetics.20
ENGINEERING EVS TO DISPLAY PROTEIN THERAPEUTICS
EVs show great potential as a vehicle for the display of biotherapeutics, such as antibodies and biological receptor proteins, both as potential agonists and as antagonistic decoy proteins, eg, for proinflammatory cytokines. Gupta et al recently showed that EVs can be engineered to simultaneously express recombinant fusion constructs consisting of domains of EV scaffold proteins fused to the extracellular domains of receptors for the proinflammatory cytokines TNF alpha and IL-6 on their surface.21 In rodent models of inflammation, these EVs were shown to exhibit potent anti-inflammatory activity greater than that clinically approved biologics targeting the TNF alpha and IL-6 signalling pathways. A similar approach has been taken to express the inflammatory cytokine IL-12 as a fusion with the EV scaffold protein PTGFRN, as a potential anti-cancer treatment.22 In this study, EVs expressing IL-12 on their surface were shown to potently reduce tumor growth following intratumoral injection. Codiak Biosciences have initiated a Phase 1 clinical trial in cutaneous T cell lymphoma with their exoIL-12 EV product (NCT05156229).
ENGINEERING EVS TO DELIVER PROTEIN CARGOES
In addition to displaying therapeutic or targeting moieties on the EV surface, many researchers have successfully used engineered EVs to deliver intraluminal protein cargoes. Scientists at Evox have developed a proprietary intein-based cleavage system to allow soluble enzymes and proteins to be luminally loaded into exosomes without a need to remain tethered to the exosomal membrane (Evox Therapeutics, manuscript in preparation). Others have demonstrated that genetically fusing target proteins to the EV scaffold protein BASP1 can result in the efficient loading and delivery of cargoes, such as ovalbumin, as well as a bacteriophage-derived RNA-binding protein and the gene editing enzyme Cas9.12 Codiak Biosciences is using this approach to develop EVs loaded with a cyclic dinucleotide (CDN) stimulator of interferon genes (STING) agonist for clinical development in solid tumors (NCT04592484). A subclass of EVs known as arrestin domain containing protein 1 (ARRDC1)-mediated microvesicles (ARMMs) has been successfully demonstrated to efficiently load (via genetic fusion to the ARRDC1 protein) and deliver a variety of therapeutic cargoes, including p53 and the CRISPR/Cas 9 guide RNA complex.23,24
EVS AS A DELIVERY VEHICLE FOR ENDOGENOUSLY LOADED RNA-BASED THERAPIES
EVs typically contain a range of RNA molecules, such as miRNA, lncRNA, and mRNA, and mRNA and their ability to deliver RNA molecules from one cell to another has be long established.25 Researchers have looked to exploit this natural nucleic acid-loading capability of EVs in order to deliver potentially therapeutic RNA payloads. Liu et al used an approach of directly transfecting siRNA molecules targeting the Mu opioid receptor (MOR), along with an engineered lamp2b construct displaying the RVG peptide into producer cells and demonstrated that the EVs produced by these cells could cross the blood-brain barrier and functionally down-regulate MOR expression.26 Moreover, they showed that the siRNA was associated with argonaute 2 (AGO2) protein within the EVs, indicating that the proteomic content of the EVs could be potentially exploited to enrich for RNA loading. At around this time, Hung and Leonard took this approach a step further, fusing Lamp2b to the RNA binding bacteriophage coat protein MS2 and engineering the cognate MS2 stem loop sequence into RNA cargoes.27 Taking this active endogenous-loading approach, which they termed Targeted and Modular EV Loading (TAMEL), they showed that RNA enrichment of up to 40-fold could be observed in EVs derived from cells transfected with this system. An alternative but conceptually similar approach developed by Martin Fussenegger and colleagues used the archaeal ribosomal protein L7Ae, which binds to the C/Dbox RNA structure, fused to the terminus of the tetraspanin CD63, coupled with the insertion of the C/Dbox structure into the 3’ UTR of target mRNA. Using this method, they could produce EVs significantly enriched for the target mRNA, as well as delivery both in vitro and in vivo.28 Recent work by Evox Therapeutics using RNA binding proteins loaded into EVs enabled > 100-fold enrichment into exosomes for mRNA containing the cognate recognition motif in its 3’ UTR (Unpublished results).
EVS AS DELIVERY VEHICLE FOR EXOGENOUSLY LOADED RNA-BASED THERAPIES
Electroporation of EVs is a well-established method for exogenously loading them with micro RNAs (miRNAs), siRNAs, and linear DNA. In a seminal study, Matthew Wood and co-workers showed that EVs that had been electroporated with siRNA targeting the Alzheimer disease (AD) target beta-secretase 1 (BACE1) could lead to significant knockdown of BACE1 expression when delivered in vivo in a mouse model of AD.15 Subsequently, it has been demonstrated that EVs electroporated with siRNA targeting oncogenic mutants of K-ras can potently suppress tumor growth in a mutant Kras-expressing human pancreatic orthotopic tumor mouse model.29
A major challenge to using electroporation to load nucleic acid therapies into EVs for clinical development is that of scaling to the large volumes needed to treat patients. Alternative approaches to loading EVs include the use of hydrophobically modified siRNAs.30 Here, Khorova and colleagues developed cholesterol- conjugated siRNAs targeting Huntingtin mRNA and showed that they could be efficiently incorporated into EVs following incubation without altering EV integrity or size distribution. Moreover, these siRNA-loaded EVs could efficiently silence Huntingtin mRNA and protein when applied to mouse primary cortical neurons. In addition, these siRNA-loaded EVs, unlike the cholesterol-tagged siRNAs alone, were able to efficiently distribute bilaterally across the mouse brain striatum.
EVS AS A METHOD OF PACKAGING ADENO-ASSOCIATED VIRUS (AAV)
Adeno-associated viruses (AAVs) are a well-established vector for gene therapy, with Luxturna (voretigene neparvovec) approved for the treatment of inherited retinal dystrophy in 2017 and Zolgensma (onasemnogene abeparvovec-xioi) approved for the treatment of spinal muscular atrophy in 2019, as well as over 100 AAV-based therapies under clinical investigation in 2021. AAV therapies still face substantial challenges, notably safety, high dosing requirements, and immunogenicity, with for example, pre-existing neutralizing antibodies against the AAV8 serotype detected in ~30% of screened patients.31 Casey Maguire and colleagues have demonstrated that AAV associated with EVs (exo-AAV) are able to be isolated through ultracentrifugation and that these exo-AAV show substantial protection from pre-existing antibodies, compared to AAV alone.32 This approach has been applied to intravitreal injection, where exo-AAV2 was observed to penetrate deeper into the retina than conventional AAV2, efficiently reaching the inner nuclear and outer plexiform.33 This approach of combining EVs with AAV is potentially a very promising method to address some of the key challenges associated with conventional therapy and may offer a path toward efficient multiple dosing of AAV therapies and the potential for lower therapeutic doses. The requirement for ultracentrifugation to prepare the exo-AAV represents a key challenge to overcome in order to appropriately scale this approach for clinical application, and so development of alternative purification and analytical techniques is likely to be essential. Another area for future development that we and others are exploring involves using EV engineering approaches to actively load AAV into EVs rather than rely solely on passive association of AAV with EVs.
FUTURE PERSPECTIVES
EVs exhibit key properties that make them extremely attractive as therapeutics, particularly their safety profile and potential for low immunogenicity. In order to effectively unlock this potential, some key challenges remain, such as the development of EV product manufacture and characterization methodologies and rapid pharmacokinetics. As discussed in this review, engineering approaches, such as the display of targeting moieties on EV scaffold proteins or the incorporation of “don’t-eat-me” signals, such as CD47 into EVs may help to surmount the challenge of rapid clearance and enhance EV biodistribution. One intriguing approach to EV therapy that could address both the manufacturing and pharmacokinetic challenges was recently reported by Fu and colleagues.34 Here, instead of using purified EVs to deliver siRNA molecules in vivo, Fu et al designed DNA vectors encoding the EV-associated protein Lamp2b fused to DNA sequences encoding for siRNAs, all under a CMV promoter. Intravenous delivery of these constructs as plasmids resulted in delivery to the liver, followed by the hepatic production of EVs encoding the siRNAs of interest. Intriguingly, the authors report substantial extrahepatic functional delivery of the siRNA to multiple tissues. By incorporating the RVG peptide into the genetic design, hepatically-generated EVs containing siRNAs designed to knockdown protein tyrosine phosphatase 1b (PTP1b), a potential obesity target associated with leptin and insulin signalling in hypothalamic neurons, were successfully delivered to the brain resulting in PTP1b knockdown and leptin and insulin sensitivity in a mouse model of obesity. This genetic delivery approach to EV production in vivo may represent a new paradigm for EV therapeutics, with the potential for a successful marriage of EV delivery of RNAi therapies with more established gene delivery approaches, such as mRNA/LNP or AAV (Figure 2). If successful, this could add substantially to our thinking around the future development of EV-based therapeutics.
REFERENCES
- Y. Couch et al., “A brief history of nearly EV-erything – The rise and rise of extracellular vesicles,” Journal of Extracellular Vesicles, vol. 10, no. 14. John Wiley and Sons Inc, Dec. 01, 2021. doi: 10.1002/jev2.12144.
- M. Yáñez-Mó et al., “Biological properties of extracellular vesicles and their physiological functions,” Journal of Extracellular Vesicles, vol. 4, no. 2015. Co-Action Publishing, pp. 1–60, 2015. doi: 10.3402/jev.v4.27066.
- J. Huotari and A. Helenius, “Endosome maturation,” The EMBO Journal, vol. 30, no. 17, pp. 3481–3500, Aug. 2011, doi: 10.1038/emboj.2011.286.
- A. E. Russell et al., “Biological membranes in EV biogenesis, stability, uptake, and cargo transfer: an ISEV position paper arising from the ISEV membranes and EVs workshop,” in Journal of Extracellular Vesicles, Dec. 2019, vol. 8, no. 1. doi: 10.1080/20013078.2019.1684862.
- A. G. Yates et al., “In sickness and in health: The functional role of extracellular vesicles in physiology and pathology in vivo: Part II: Pathology,” Journal of Extracellular Vesicles, vol. 11, no. 1. John Wiley and Sons Inc, Jan. 01, 2022. doi: 10.1002/jev2.12190.
- K. Meehan and L. J. Vella, “The contribution of tumour-derived exosomes to the hallmarks of cancer.,” Crit Rev Clin Lab Sci, vol. 53, no. 2, pp. 121–31, 2016, doi: 10.3109/10408363.2015.1092496.
- G. Chen et al., “Exosomal PD-L1 contributes to immunosuppression and is associated with anti-PD-1 response,” Nature, vol. 560, no. 7718, pp. 382–386, Aug. 2018, doi: 10.1038/s41586-018-0392-8.
- B. B. Guo, S. A. Bellingham, and A. F. Hill, “Stimulating the Release of Exosomes Increases the Intercellular Transfer of Prions.,” J Biol Chem, vol. 291, no. 10, pp. 5128–37, Mar. 2016, doi: 10.1074/jbc.M115.684258.
- S. Gurung, D. Perocheau, L. Touramanidou, and J. Baruteau, “The exosome journey: from biogenesis to uptake and intracellular signalling,” Cell Communication and Signaling, vol. 19, no. 1. BioMed Central Ltd, Dec. 01, 2021. doi: 10.1186/s12964-021-00730-1.
- Z. Andreu and M. Yáñez-Mó, “Tetraspanins in extracellular vesicle formation and function,” Frontiers in Immunology, vol. 5, no. SEP, 2014, doi: 10.3389/fimmu.2014.00442.
- D.-K. Kim, J. Lee, R. J. Simpson, J. Lötvall, and Y. S. Gho, “EVpedia: A community web resource for prokaryotic and eukaryotic extracellular vesicles research.,” Semin Cell Dev Biol, vol. 40, pp. 4–7, Apr. 2015, doi: 10.1016/j.semcdb.2015.02.005.
- K. Dooley et al., “A versatile platform for generating engineered extracellular vesicles with defined therapeutic properties,” Molecular Therapy, vol. 29, no. 5, pp. 1729–1743, May 2021, doi: 10.1016/j.ymthe.2021.01.020.
- M. Morishita, Y. Takahashi, M. Nishikawa, and Y. Takakura, “Pharmacokinetics of Exosomes-An Important Factor for Elucidating the Biological Roles of Exosomes and for the Development of Exosome-Based Therapeutics.,” J Pharm Sci, vol. 106, no. 9, pp. 2265–2269, 2017, doi: 10.1016/j.xphs.2017.02.030.
- R. Huey, S. Hawthorne, and P. McCarron, “The potential use of rabies virus glycoprotein-derived peptides to facilitate drug delivery into the central nervous system: a mini review.,” J Drug Target, vol. 25, no. 5, pp. 379–385, Jun. 2017, doi: 10.1080/1061186X.2016.1223676.
- L. Alvarez-Erviti, Y. Seow, H. Yin, C. Betts, S. Lakhal, and M. J. A. Wood, “Delivery of siRNA to the mouse brain by systemic injection of targeted exosomes,” Nature Biotechnology, vol. 29, no. 4, pp. 341–345, Apr. 2011, doi: 10.1038/nbt.1807.
- X. Liu et al., “Overcoming the blood-brain barrier by using a multistage exosome delivery system to inhibit central nervous system lymphoma.,” Nanomedicine, vol. 41, p. 102523, 2022, doi: 10.1016/j.nano.2022.102523.
- X. Shi et al., “Genetically Engineered Cell-Derived Nanoparticles for Targeted Breast Cancer Immunotherapy,” Molecular Therapy, vol. 28, no. 2, pp. 536–547, Feb. 2020, doi: 10.1016/j.ymthe.2019.11.020.
- D. Pilati and K. A. Howard, “Albumin-based drug designs for pharmacokinetic modulation.,” Expert Opin Drug Metab Toxicol, vol. 16, no. 9, pp. 783–795, Sep. 2020, doi: 10.1080/17425255.2020.1801633.
- S. Kamerkar et al., “Exosomes facilitate therapeutic targeting of oncogenic KRAS in pancreatic cancer,” Nature, vol. 546, no. 7659, pp. 498–503, Jun. 2017, doi: 10.1038/nature22341.
- N. Parada, A. Romero-Trujillo, N. Georges, and F. Alcayaga-Miranda, “Camouflage strategies for therapeutic exosomes evasion from phagocytosis,” Journal of Advanced Research, vol. 31. Elsevier B.V., pp. 61–74, Jul. 01, 2021. doi: 10.1016/j.jare.2021.01.001.
- D. Gupta et al., “Amelioration of systemic inflammation via the display of two different decoy protein receptors on extracellular vesicles.,” Nat Biomed Eng, vol. 5, no. 9, pp. 1084–1098, 2021, doi: 10.1038/s41551-021-00792-z.
- N. D. Lewis et al., “Exosome surface display of IL12 results in tumor-retained pharmacology with superior potency and limited systemic exposure compared with recombinant IL12,” Molecular Cancer Therapeutics, vol. 20, no. 3, pp. 523–534, Mar. 2021, doi: 10.1158/1535-7163.MCT-20-0484.
- Q. Wang, J. Yu, T. Kadungure, J. Beyene, H. Zhang, and Q. Lu, “ARMMs as a versatile platform for intracellular delivery of macromolecules,” Nature Communications, vol. 9, no. 1, Dec. 2018, doi: 10.1038/s41467-018-03390-x.
- H. Choi et al., “Exosome-based delivery of super-repressor IκBα relieves sepsis-associated organ damage and mortality.,” Sci Adv, vol. 6, no. 15, p. eaaz6980, 2020, doi: 10.1126/sciadv.aaz6980.
- L. Cheng and A. F. Hill, “Therapeutically harnessing extracellular vesicles,” Nature Reviews Drug Discovery, Mar. 2022, doi: 10.1038/s41573-022-00410-w.
- Y. Liu et al., “Targeted exosome-mediated delivery of opioid receptor Mu siRNA for the treatment of morphine relapse.,” Sci Rep, vol. 5, p. 17543, Dec. 2015, doi: 10.1038/srep17543.
- M. E. Hung and J. N. Leonard, “A platform for actively loading cargo RNA to elucidate limiting steps in EV-mediated delivery.,” J Extracell Vesicles, vol. 5, p. 31027, 2016, doi: 10.3402/jev.v5.31027.
- R. Kojima et al., “Designer exosomes produced by implanted cells intracerebrally deliver therapeutic cargo for Parkinson’s disease treatment,” Nature Communications, vol. 9, no. 1, Dec. 2018, doi: 10.1038/s41467-018-03733-8.
- M. Mendt et al., “Generation and testing of clinical-grade exosomes for pancreatic cancer,” JCI Insight, vol. 3, no. 8, Apr. 2018, doi: 10.1172/jci.insight.99263.
- M. C. Didiot et al., “Exosome-mediated delivery of hydrophobically modified siRNA for huntingtin mRNA silencing,” Molecular Therapy, vol. 24, no. 10, pp. 1836–1847, Oct. 2016, doi: 10.1038/mt.2016.126.
- S. J. Aronson et al., “Prevalence and Relevance of Pre-Existing Anti-Adeno-Associated Virus Immunity in the Context of Gene Therapy for Crigler-Najjar Syndrome.,” Hum Gene Ther, vol. 30, no. 10, pp. 1297–1305, 2019, doi: 10.1089/hum.2019.143.
- B. György, Z. Fitzpatrick, M. H. W. Crommentuijn, D. Mu, and C. A. Maguire, “Naturally enveloped AAV vectors for shielding neutralizing antibodies and robust gene delivery invivo,” Biomaterials, vol. 35, no. 26, pp. 7598–7609, 2014, doi: 10.1016/j.biomaterials.2014.05.032.
- S. J. Wassmer, L. S. Carvalho, B. György, L. H. Vandenberghe, and C. A. Maguire, “Exosome-associated AAV2 vector mediates robust gene delivery into the murine retina upon intravitreal injection.,” Sci Rep, vol. 7, p. 45329, 2017, doi: 10.1038/srep45329.
- Z. Fu et al., “In vivo self-assembled small RNAs as a new generation of RNAi therapeutics,” Cell Research, vol. 31, no. 6, pp. 631–648, Jun. 2021, doi: 10.1038/s41422-021-00491-z.
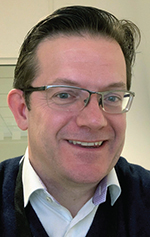
Dr. David Lowe is Vice President, Research, at Evox Therapeutics, and has more than 20 years of biotech and large pharma R&D experience in advancing protein and antibody-based therapeutics as well as exploring novel therapeutic modalities, such as gene therapy, mRNA therapeutics, and exosomes. Prior to his joining Evox, he spent more than 12 years at AstraZeneca, most recently as Senior Director in charge of Biologics Engineering.
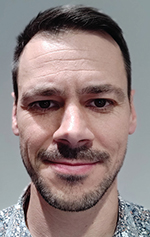
Dr. Justin Hean has spent more than a decade within the exosomal field, having spent half that time developing with Evox Therapeutics, making him one of Evox’s longest-serving staff members. He has particular interest in the alteration of exosomes and their production for therapeutic benefit. Currently, he leads a team exploiting facets of exosome engineering, including luminal loading of beneficial payload and surface decoration of functional ligands.
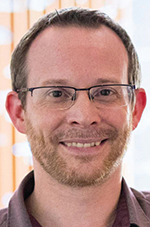
Dr. Dave Carter has more than 15 years of leading multidisciplinary research projects in the exosome field. As a Professor in Biomedical Science at Oxford Brookes University, he secured over £1m in grant funding, published more than 50 papers, and served as the first President of the UK Society for Extracellular Vesicles, before joining Evox in 2021 as Research Director in Exosome Engineering.
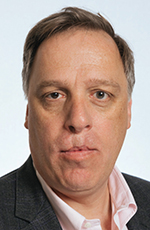
Dr. Antonin de Fougerolles is Chief Executive Officer of Evox Therapeutics, and has more than 20 years of biotech R&D experience in building out drug pipelines. He has played a key role in developing and successfully advancing three new drug modalities toward the market and in helping build several multi-billion dollar companies from the start-up stage, including Moderna, Alnylam, and Ablynx.
Total Page Views: 5461