Issue:March 2024
ENGINEERING BIOLOGY - Scaling Engineering Biology to Accelerate Advances in Healthcare
INTRODUCTION
In recent years, significant progress in engineering biology has transformed the world of healthcare, providing researchers with revolutionary biological tools to develop novel therapeutics. From monoclonal antibody therapies — the fastest growing area of biotherapeutics — to mRNA vaccines, CAR-T cell therapies, and drug biosynthesis, engineering biology is providing solutions to some of the biggest challenges in healthcare.
The potential of this transformative technology to support global healthcare challenges was demonstrated during the response to the COVID-19 pandemic, facilitating the rapid production of diagnostic tests, preventative vaccines, and therapies. The pre-existence of biofoundries — facilities containing high-throughput bioengineering and robotic capabilities — enabled engineering biology to be effective during the response to the pandemic, scaling global testing and vaccine manufacture.1
In part, due to the sheer scale of resources recruited to tackle the pandemic, vaccine development was incredibly quick compared with the development of traditional vaccine technologies, which on average spans 10 years, accounting for preclinical, Phase 1–3 testing, filing, and registration. In contrast, once the SARS-CoV-2 sequence was released, it only took 11 months for the first COVID-19 vaccine to be approved by regulatory bodies in the UK, US, and Europe.2
However, numerous healthcare challenges exist beyond COVID-19. The extensive list of conditions engineering biology is poised to address includes cancer, neurodegenerative diseases, infectious diseases, and many others. But how can we tackle all these challenges at pace and scale when it took the collective efforts of the world’s biotechnology and pharmaceutical industries to conquer just one?
While engineering biology has already proven to be transformational to some of healthcare’s greatest challenges, we simply don’t yet have access to all the tools to benefit from it at the scale required.
TIME & SCALE IN HEALTHCARE
While the need for speed and scale is obvious in the context of the recent pandemic response, the everyday relevance of these factors is understood acutely within the pharmaceutical industry. Cost, speed, and quality are essential elements for enabling leading pharmaceutical companies to stay ahead of the curve. Each has been improved by applying principles from engineering biology, but there is great potential to further optimize workflows that could, in turn, deliver life-saving treatments to patients faster and at lower prices.3
Advances in the treatment of hematologic malignancies represent an example of how improved workflows can impact patient outcomes. Engineering biology played a significant role in the development of CAR-T cell immunotherapy, which uses novel cell therapy and genetic reprogramming methods to genetically alter patients’ T cells, reprogramming them to target the patients’ tumor cells.4 The first CAR-T cell therapy was approved for the treatment of previously incurable hematologic cancers in 2017. Six CAR-T cell therapies have since been approved by the US Food and Drug Administration (FDA), costing over $350,000 per infusion — a cost largely associated with laborious and complex manufacturing processes. Implementing innovative technologies to scale and optimize CAR-T cell production may help improve access and meet the increasing demand for patients with cancer.5
THE NEED FOR IMPROVED GENE SYNTHESIS
Engineering biology workflows follow an iterative Design-Built-Test-Learn (DBTL) methodology. To assess the capabilities of the DBTL cycle, the US Department of Advanced Research Projects Agency administered a “pressure test” to evaluate a biofoundry, where the team was given 90 days to generate organisms that would produce 10 molecules.6 Despite the identities of the molecules being unknown in advance, 6 out of 10 targets were successfully generated during the performance period. However, sourcing DNA was the major bottleneck, accounting for around half the allotted time.
This bottleneck is poised to become much more acute with the advancement of artificial intelligence (AI) in protein design. Scientists now have access to powerful tools to facilitate the discovery of de novo structures with useful functions, such as improved stability or new catalytic activities.7 To engineer these functions, specific mutations may be introduced and tested in iterative cycles. Machine learning (ML)-based methods using combinatorial libraries of mutations create opportunities to investigate sequence space more efficiently and screen protein variants rapidly in silico.8 As these programs continue to expand, the need for DNA to iteratively test these sequences and reap their benefits will expand enormously.
TECHNOLOGICAL CHALLENGES FOR DNA AVAILABILITY
Despite the evident need, increasing access to gene-length DNA isn’t straightforward. Numerous innovations in DNA sequencing technology have been made, but the barriers to the synthesis of long and complex DNA sequences at scale need to be overcome to drive engineering biology forward.9
Efficiency of the elongation cycle is one of several factors contributing to the challenge of producing gene-length DNA.10 With increasing DNA length, the yield of error-free DNA decreases significantly. For example, with an elongation cycle efficiency of 99%, the theoretical yield calculation for an oligo composed of 120 bases is as follows: (0.99120 × 100%) = 30%.9 The yield for an oligo of 200 bases, however, decreases to 13%, and increasing the DNA length to 1 kilobase causes the yield to plummet to less than 0.01%.
For sequences longer than 1 kilobase, DNA is assembled from a pool of synthetic oligonucleotides, leading to increased challenges associated with the time and cost of oligo assembly and error correction. This process is error-prone, and validating a sequence becomes more time-consuming as the length of the DNA product increases (Figure 1).
Screening for the correct product generally involves cloning into a vector, transformation into bacteria, isolation of individual clones, and validation of the sequence by Sanger sequencing. This method is simple and efficient for sequences of up to 300 base pairs; however, the probability of error increases significantly with increasing DNA length. Many clones must be generated and sequenced to obtain the correct product, adding to the time and costs required for DNA synthesis.
Current DNA synthesis methods are effective for the synthesis of short DNA sequences, but new technologies are critical to enabling parallel synthesis of many sequences, assembly of gene-length DNA, and error correction via built-in programs.
BENCHTOP GENE SYNTHESIS
The development of benchtop DNA printers represents a new breakthrough in DNA synthesis technologies and a potential solution to the growing demand for this process to become more affordable, flexible, and scalable than is currently available through service labs (Figure 2).11
Having an instrument capable of synthesizing and assembling gene-length DNA, correcting errors, and producing large quantities of sequences in parallel will simplify and accelerate each iteration of a complex experiment, improving access to long, accurate DNA sequences and promoting unprecedented speed and control of synthesis. Current benchtop DNA printing is limited to shorter DNA strands. As errors increase exponentially with the length of DNA, developing a benchtop machine to synthesize error-free long DNA requires the re-imagination of DNA synthesis technology.
THERMAL SEMICONDUCTOR CHIP TECHNOLOGY BRINGING GENE SYNTHESIS TO EVERY LAB
Innovative technologies to address the limitations of current benchtop DNA printing have recently emerged, bringing researchers closer to accessing gene-length, error-free DNA synthesis.
For example, recent microarray formats enable parallel synthesis of sequences at distinct reaction sites, with semiconductor chips greatly increasing multiplexing capabilities for DNA synthesis. Combining highly parallel synthesis on a silicon chip with precise, thermal control of DNA synthesis increases the control and accuracy of DNA synthesis (Figure 3).
Re-engineering DNA synthesis chemistry can allow for selective elongation at specific synthesis sites via temperature-sensitive protecting groups at the sequence termini.12 After the initiation of an elongation cycle, nucleotides will only be added to chains present at heated reaction sites, enabling precise control over the synthesis of thousands of heterogeneous sequences in parallel.13
Methods are also being developed to integrate DNA synthesis with a staged assembly and error-removal process. For example, the Binary Assembly® process joins complementary DNA strands by selective transfer of DNA from synthesis sites to assembly sites on silicon chips.14 After complementary strands are annealed, the assembly sites are heated to sequence-dependent temperatures that promote rapid dissociation of imperfect matches from the chip, thereby separating and removing error-containing sequences whilst maintaining those with correct homology during the assembly process.14 This offers significant advantages over conventional approaches by lowering error rates and eliminating time-consuming post-synthesis steps.12 Duplex DNA fragments can be joined, and the process is repeated to assemble gene-length sequences.
Moreover, thermal control at distinct sites combined with parallel synthesis capabilities may also facilitate the elongation of challenging DNA regions. For example, selective heating can promote the synthesis of DNA segments with high GC content, which have higher melting temperatures and are prone to secondary structure formation.10
PROVIDING A LEAD TIME ADVANTAGE
The integration of thermally controlled semiconductor gene synthesis technology with a built-in error removal process into a benchtop platform will greatly expand the capabilities for the rapid synthesis of accurate gene-length DNA, reducing error rates and eliminating time-consuming post-synthesis steps.
Access to accurate gene-length DNA poses a barrier in bioengineering research, as a centralized approach to gene synthesis can limit the DBTL cycle and conventional synthesis technologies are unable to meet the demand for long and complex sequences.
Overcoming such limitations through the development of a benchtop synthesis platform has the potential to expedite the discovery and development time for drugs and biotherapeutics, greatly accelerating the rate at which engineering biology can shape the future of healthcare.
REFERENCES
- Vickers, C. E. & Freemont, P. S. Pandemic preparedness: synthetic biology and publicly funded biofoundries can rapidly accelerate response time. Nat Commun 13, 453, doi:10.1038/s41467-022-28103-3 (2022).
- Agrawal, G., Hermann, R., Poetes, R., Steinmann, M. & Møller, M. Fast-forward: Will the speed of COVID-19 vaccine development reset industry norms? (2021). <https://www.mckinsey.com/industries/life-sciences/our-insights/fast-forward-will-the-speed-of-covid-19-vaccine-development-reset-industry-norms>.
- David, F. et al. A Perspective on Synthetic Biology in Drug Discovery and Development-Current Impact and Future Opportunities. SLAS Discov 26, 581-603, doi:10.1177/24725552211000669 (2021).
- Porter, D. L., Levine, B. L., Kalos, M., Bagg, A. & June, C. H. Chimeric antigen receptor-modified T cells in chronic lymphoid leukemia. N Engl J Med 365, 725-733, doi:10.1056/NEJMoa1103849 (2011)
- Abou-El-Enein, M. et al. Scalable Manufacturing of CAR T cells for Cancer Immunotherapy. Blood Cancer Discov 2, 408-422, doi:10.1158/2643-3230.BCD-21-0084 (2021).
- Casini, A. et al. A Pressure Test to Make 10 Molecules in 90 Days: External Evaluation of Methods to Engineer Biology. J Am Chem Soc 140, 4302-4316, doi:10.1021/jacs.7b13292 (2018).
- Eisenstein, M. AI-enhanced protein design makes proteins that have never existed. Nat Biotechnol 41, 303-305, doi:10.1038/s41587-023-01705-y (2023).
- Wu, Z., Kan, S. B. J., Lewis, R. D., Wittmann, B. J. & Arnold, F. H. Machine learning-assisted directed protein evolution with combinatorial libraries. Proc Natl Acad Sci U S A 116, 8852-8858, doi:10.1073/pnas.1901979116 (2019).
- Hoose, A., Vellacott, R., Storch, M., Freemont, P. S. & Ryadnov, M. G. DNA synthesis technologies to close the gene writing gap. Nat Rev Chem 7, 144-161, doi:10.1038/s41570-022-00456-9 (2023).
- Carbonell, P., Radivojevic, T. & Garcia Martin, H. Opportunities at the Intersection of Synthetic Biology, Machine Learning, and Automation. ACS Synth Biol 8, 1474-1477, doi:10.1021/acssynbio.8b00540 (2019).
- Fernández, C. R. DNA synthesis: tackling the main bottleneck in biology research. (2022). <https://www.labiotech.eu/in-depth/dna-synthesis-biology-research/>.
- Crosby, S. Unlocking synthetic biology through DNA synthesis. Chimica Oggi – Chemistry Today 38, 22-24 (2020).
- Ferguson, A. J. et al. Thermofluidic chip containing virtual thermal wells. Engineering Biology 3, 20-23, doi:10.1049/enb.2018.5010 (2019).
- Evonetix Granted Patent for Binary Assembly Method for Gene Synthesis. (2022). <https://www.businesswire.com/news/home/20220509005406/en/Evonetix-Granted-Patent-for-Binary-Assembly-Method-for-Gene-Synthesis>.
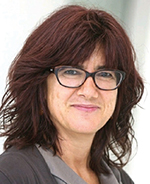
Raquel Sanches-Kuiper is Vice President of Science and Applications at Evonetix, with a background in Protein Engineering and Product Development, including the early development of NGS technologies at Solexa and Illumina.
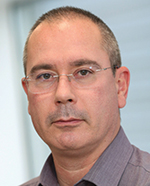
Dr. Matthew Hayes is CTO and Founder of Evonetix with a background in Electronic Engineering and multi-disciplinary system design. He was previously Head of Technology for the Global Medtech Division of Cambridge Consultants.
Total Page Views: 2629