Issue:January/February 2014
ADVANCED DELIVERY DEVICES - Engineering the Perfect Click for Drug Delivery Devices
If you were to tell some people that one of the most frustrating aspects of the development of a drug delivery device would be the little clicks that it makes as part of its operation, they would probably think you had lost your sense of priority. Yet time and time again, the engineering of the click becomes a real and serious issue. Device developers know this – whether human factors experts or industrial designers, mechanical engineers, or risk analysis teams – yet this aspect of device design, like many others, is frequently not given the attention it deserves. So why is a click so important?
For many drug delivery devices, including inhalers but particularly pen and autoinjectors, the feedback users receive from a device during the sequence of use can be critical in helping them achieve effective and complete delivery of their medication. Designers will endeavour to make device use as intuitive as possible, but the products that we are considering are used by people from all walks of life, of all ages and physical condition, so intuitiveness is not always easy to achieve. Hence, feedback from
the device can help give the user confidence that they are carrying out the steps required correctly, especially when these steps are part of the instructions for use and, if appropriate, patient training.
WAIT FOR THE CLICK…
Take the Asmabec® Clickhaler® for example, a dry powder inhaler for asthma treatment. The IFU instructs the user to “press the dosing button down firmly once until it clicks, then release.” Or the Aranesp® SureClick® autoinjector IFU, which references
first and second clicks, with the instruction to “Wait until the second click before lifting the injector from the injection site.” And then there is the ClikSTAR® Lantus® for injection of insulin – “screw in the insulin cartridge holder until it clicks into place…” Or, as a final example, the Advair® Diskus® – the current world best seller – which has three basic instruction steps: “Open, Click, Inhale.” All these instruction steps are critical to achieving successful delivery of a full dose, and all are dependent on the user hearing and correctly interpreting the click.
THE CLUE IS IN THE NAMES…
So clicks are vitally important for users, and yet we see time and again they are not good enough. Engineers and designers face this issue frequently, and there is a wealth of evidence from user research – formative and summative – that highlights not only the criticality of these audible cues and how they combine with other aspects of interaction design to influence user behavior, but also what happens when clicks are not right. Subtle variations can mean the difference between success and failure when self-administering vital medication, and as there won’t always be a second chance, the user must be certain that they have received the medication they need. Yet clicks are different to other critical characteristics of device performance, such as actuation forces or dose volumes, in that they are not so easy to define and describe. We know what we want when we hear it – and we know what we don’t want as well – but how can we describe clicks accurately? Maybe we should start with defining what a good click “looks” like?
VISUALIZING THE PERFECT CLICK
It is one thing to recognize the importance of a device performance characteristic, but quite another to know how to engineer it; to know how to develop, optimize, and verify a characteristic so that we get what we need, when we need it, every time.
We can (usually) describe quite easily, in subjective terms, what we want from the click that a device has to make. For example, we can say that it needs to be clear, distinct, gentle, or that it needs to give an indication of quality, robustness, or re-assurance. And it certainly mustn’t be scary or alarming. We know what we mean and it is certainly a good idea to think in these terms to begin with. But to really get into the detail, we need to establish a more objective set of descriptors that allow us to define good and bad clicks.
A good – and fairly obvious – starting point is to look at a click’s acoustic waveform. This is the best way to characterize a sound, after all, and that is primarily what a click is. With high-quality recording equipment and software, it is relatively straightforward to obtain a high-resolution acoustic signal, in terms of amplitude versus time. But care must be taken when recording the sounds, especially if you wish to exclude external (consequential) sounds such as reverberation. If you want to isolate the click fully from all external in mind though, that a click heard in a chamber is not how the user will hear it. Similar consideration needs to be given to the way you hold the device, as quite often the caseworks will act as soundboards.
Once you have obtained the acoustic waveform (Figure 2, which shows a typical waveform for a device click), you can then apply a range of smoothing and clipping algorithms to give an amplitude envelope that can be characterized for each of the click’s two main stages – attack and decay – as shown in Figures 3 and 4. This approach is a little different to applying a standard ADSR (Attack, Decay, Sustain, and Release) envelope as might be done in other acoustic analyses. However, clicks are by their nature very short acoustic events, and if we can reduce the level of detail while retaining the level of characterization needed to work effectively, that is generally a good thing.
Once completed, the characterization of the acoustic waveform will result in a set of parameters that can be used to describe each click in a simplified but more manageable way. This opens up opportunities for specifying (and hence also verifying) clicks, for comparing different clicks in order to improve or optimize them, or for comparing the consistency of the same click, perhaps under different conditions, such as hot or cold temperatures.
As well as looking at the amplitude waveform of the sound, it is also possible to use Fast Fourier Transform analysis to obtain a spectrum of sound frequencies that make up the click. Off-the-shelf software packages can carry out this analysis very quickly and effectively (Figure 5 shows a screen capture from the Audacity software package, which is one possible tool). As for the amplitude waveform, these outputs can then be clipped and smoothed as appropriate to give a more readily applicable tool when seeking to characterize the click’s acoustic signature.
How much detail is required when capturing and analyzing the sound characteristics of the device will vary on a case-by-case basis. The point here is to show that, through careful application of reasonably accessible tools and methodologies, important acoustic device characteristics can be readily described, analyzed, and understood.
WHAT INFLUENCES CLICK CHARACTERISTICS?
When considering click characteristics, the first thing to determine is what is actually making the click, which might not be what you think. A typical click will involve a deflecting member with a relatively sharp tipped form somewhere on its length; this rides over a step or falls off an edge, which causes it to snap back. In some embodiments, the substantial part of the click will be generated by this deflected member returning quickly and striking a surface, but in many cases, it is the action of the tip falling off the edge – so that the member accelerates through the air – which generates the sound energy. This is further combined, to differing degrees, with vibration of the edge feature itself (and any features that support it) as these are also likely to vibrate to some extent due to the rapid removal of the reaction force.
Because sound is a very low-energy phenomenon, it only takes small variations in system properties, such as feature stiffness, inertia, additional system vibrations (for example a rattle), or damping, to result in very different sounds. Hence, material properties (modulus, density) feature design, joint characteristics (if the click features are part of an assembly), and how the device is held or secured can all influence the characteristic. When developing a set of click features, all of these elements need to be considered, just as when investigating an unwanted click in an existing system. In the latter case, it may also be worth using high-speed video – possibly synchronized with acoustic measurements – to establish exactly what is happening at the instant of the click. Things may not be what they seem…
HOW REPEATABLE WILL THE CLICK BE?
Any variation in the design parameters that influence the system properties previously described will clearly result in a variation in the click that we hear. Some parameters will be relatively constant once the design specification is finalized, but others can also vary through the lifecycle of device use.
The impact of temperature on material – and hence feature stiffness – needs to be considered, and material selection will clearly be a driving factor. Material suppliers DuPont™, for example, provides information on which of its polymer grades might be most appropriate for a particular application, and provide an interesting case study on their Delrin® 100ST super tough grade used within the automotive industry. It is selected for a combination of properties, including toughness and flex fatigue at temperature extremes in order to ensure the required auditory safety signal (in other words, the click) is achieved on replacement of a fuel filler cap.
This automotive example may be an extreme case in terms of possible temperature of use (the material is approved for use from -40°C to +80°C), but it is easy to envisage scenarios in which the environment of use of a medical device, though not as extreme, is either difficult to control (for example, the emergency use inhaler or injector) or different to the conditions anticipated by the designer (such as a device used straight after removal from a fridge, where it is stored due to the nature of the drug formulation). Also, the nominal form and stiffness of the key features may not remain constant throughout device life; permanent strain may arise from over-stressing, fatigue (due to repeated use or play by the user), or creep, which can occur if the design allows the main click features to be left in a deflected, stressed position for significant periods of time.
Engineering analysis can be of assistance here and, for these examples, finite element analysis is an effective way to develop system understanding. For example, changes in material modulus arising from temperature changes allow assessment of the impact on
contact forces (which relate to click energy), while a review of peak strains and stresses (see Figure 6 for an example of an FEA study) across the range of anticipated deflections will inform review of susceptibility of the design to permanent deformation or creep.
Another aspect of variation inherent to a design is the manufacturing tolerance that can be achieved, and tolerance analysis is an indispensable tool for assessing these potential effects. We all remember that the contact force for a deflected member (of the kind shown in Figure 6) varies with the cube of the section thickness, but understanding the combined impact of all likely variations – including tolerances on diameters, ovality, or concentricity – is less intuitive though readily achievable with a bit of basic MathCAD.
GOOD CLICKS – A QUESTION OF TIMING
Where tolerance analysis can also prove invaluable is in the prediction of variation in the timing of a click. Going right back to the use of clicks as a reference point in an IFU or PIL, if we are asking the patient to react to the generation of a click from a device, we need to be sure the click happens at the right time. This can be critical if the click is linked to mechanisms that may influence dose cutting or dose delivery, engagement/release of safety features, or successful location/retention of a key element of the device. For such critical features of a design, it is usually necessary to build in a safety factor so that, allowing for system variations, the click will always happen before or after (depending on the case in question) the critical device state is achieved. Tolerance analysis then allows the design engineer to assess what happens at the other end of the system variance – how early or late – the click might occur, and what the implication might be. Figure 7 shows a very basic tolerance stack that illustrates potential angular misalignment in the timing of two diametrically opposite clicks which, in theory, should happen at the same time, but in practice will probably not. By building up a tolerance analysis in a mathematical package, such as MathCAD, rather than in a spreadsheet, it is comparatively straightforward to introduce more complex functions and inter-relationships. This allows us to take into account complex trigonometry or system dynamics, such as spring and frictional forces, and hence achieve a much more comprehensive picture of a system and its sensitivities and variations. Through use of statistical methods, such as Monte Carlo analyses, and input data, including desired and/or achieved process capabilities, a full picture of the timing of a click relative to other key mechanism functions can be derived for a range of manufactured devices real or virtual.
CHOOSE YOUR WEAPONS….
This article has outlined a range of tools and techniques for objectively describing many of the characteristics of a highly subjective, but often important and overlooked, device performance requirement – the click. Not all of this analysis is needed every time a click is required or desired, but being aware of these options and how they can be applied during the development process (not during design verification and validation) puts the designer in a stronger position. Device testing – from prototypes to pilot devices, in the lab and in the hands of users – is of course another key part of developing an effective click, but to rely solely on testing without building an understanding of how the click is working risks delays, through poorly guided design iteration, and potential compromise on performance.
Yet, to reiterate what was said in the introduction, compromise may simply not be an option. The need to engineer designs that pose a minimum risk of use-error is one of the hot issues in medical device development at the moment – a key focus of the US and European regulatory bodies whose approval for product launch is required. All aspects of user-device interaction can be critical to success in this regard, including the humble click, and more and more people in the industry are recognizing and learning this, sometimes the hard way. And the hard way can be very hard indeed.
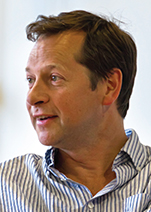
Chris Hurlstone is currently the Director of Engineering for Team Consulting, directing the company’s engineering expertise and working with the heads of development groups to ensure Team delivers consistently world-class consultancy services and robust, reliable, user-focused, and commercially successful device solutions. He is still very much hands-on, especially in areas such as risk management, technical audit, design verification, industrialization, and technical troubleshooting. Mr. Hurlstone has more than 15 years of experience with Team, developing technologies and devices for healthcare markets. He has successfully brought products to market in technical lead and project management roles, including inhalers, injectors, and an award-winning ophthalmoscope, and in the process, has worked extensively with global suppliers of manufacturing and technical expertise. A named inventor on numerous patents, he has a strong track record in delivering innovative and robust solutions to many of Team’s international clients. Mr. Hurlstone graduated from the University of Cambridge with a degree in Mechanical Engineering and a post-graduate diploma in Design, Manufacture, and Management.
Total Page Views: 2705