Issue:September 2024
SILICON-STABILIZED HYBRID LNPS - Next-Generation Delivery of RNA Therapeutics
INTRODUCTION
In recent years, lipid nanoparticles (LNPs) have revolutionized the field of nucleic acid therapeutics by overcoming significant challenges in the cellular delivery of DNA and RNA. They particularly rose to prominence as the mRNA delivery technology in the Moderna and Pfizer/BioNTech COVID-19 vaccines, but they are also being applied in over 200 ongoing clinical trials of other RNA-based drugs.1-4
Despite these impressive successes, current LNP formulations face some recognized limitations that are critical to overcome if we are to see improved clinical translation of RNA-based therapeutics.5 These can be briefly summarized as stability, safety, targeting, and transfection efficiency. The following sections will consider these current shortcomings, as well as some accompanying manufacturing challenges, and will show how they can be addressed by SiSaf’s silicon-stabilized hybrid LNPs (sshLNPs), marketed under the trade name of Bio-Courier.®
LNPS & SSHLNPS
In general, the LNPs used to deliver nucleic acid therapeutics consist of four key components: ionizable or cationic lipids, helper lipids, PEGylated lipids, and cholesterol (or a related compound). A general schematic illustrating the role of each of these components is shown in Figure 1. Importantly, the exact nature of the constituents used in a given LNP formulation will define its physical, chemical, pharmacokinetic, and pharmacodynamic properties.
In recent years, intensive research has enabled fine-tuning of these characteristics for various therapeutic contexts, but to date, only a small subset of reported components has been licensed for use in drug delivery formulations.6 Considerable optimization work may also be necessary, as illustrated by the case of patisiran (the first FDA-approved siRNA therapy delivered using LNPs), in which over 300 ionizable lipids were screened in developing the final formulation.7
Bio-Courier sshLNPs combine organic lipids with inorganic hydrolyzable silicon (Figure 2). The silicon matrix stabilizes both the lipid components and the RNA payload, reducing or completely removing the need for ionizable or cationic lipids, PEGylated lipids, or cholesterol.8-11 As we will show, this addresses some of the safety concerns associated with current LNP formulations and can also improve targeting and transfection.
KEY CHALLENGE #1: STABILITY
The stability of LNPs inevitably impacts the efficacy and safety of the administered therapy. It depends on both the overall structural integrity of the particles and the chemical stability of the individual constituents, either in storage or upon exposure to biological fluids. The large-scale international rollout of COVID-19 mRNA vaccines highlighted some obvious shortcomings of existing technologies for clinical application of LNPs, especially in the requirement for storage at ultralow temperature and the limited shelf life.12,13
Typically, LNPs and liposomes incorporate lipid and phospholipid components that contain ester linkages. These are prone to chemical hydrolysis (particularly under acidic or basic conditions), meaning ester hydrolysis can be a limiting factor for stability and shelf-life of lipid-lipid systems stored in aqueous solution.14 Ester linkages are also prone to enzymatic degradation in vivo by esterases, as shown in a recent pharmacokinetic study by Moderna that investigated the metabolic fate of “Lipid 5”, a commonly used ionizable lipid component in LNPs for preclinical studies.15 In contrast, Bio-Courier technology is able to decrease chemical hydrolysis of ester linkages in lipid constituents via silicon-lipid binding (Figure 2).
Relatedly, a notable study by Packer et al identified inactivating lipid-RNA adducts in COVID 19 mRNA vaccines, formed via oxidation of the ionizable lipid component followed by reaction with the RNA.16 The proposed mechanism could apply to all LNPs containing ionizable lipids, and as a result, testing for these impurities is expected to be incorporated into future QC protocols.17 Other investigators have observed significantly lower protein expression after exposure of mRNA-loaded LNPs to fluorescent lighting, suggesting photochemical degradation of the encapsulated RNA under conditions that may be relevant to clinical practice. These findings, together with other unanswered questions regarding the current formulations, have naturally accelerated the search for improved technologies. In an important recent example, Meulewaeter et al described the development of a novel lyophilized LNP formulation that maintained in vivo mRNA transfection efficiency after 12 weeks at room temperature.17-20
Superior physical stability is a core feature of Bio-Courier sshLNPs. Unlike LNPs formulated without silicon, they maintain their original polydispersity index (PDI) after multiple cycles of high shear force extrusion that induce repeated mechanical stress. In addition, sshLNPs preserve their original zeta potential for at least 6 months at room temperature (indicating surface structural stability), whereas a significant decrease was seen for standard LNPs after only four weeks under the same conditions. There is also evidence for improved RNA protection with sshLNPs. In one such test, a representative Bio-Courier formulation was able to fully protect mRNA against degradation in bovine serum for at least 24 h at 37°C (Figure 3).
KEY CHALLENGE #2: SAFETY & ADVERSE REACTIONS
It is vital to ensure the encapsulated RNA does not leak from LNPs into the systemic circulation. This would cause unwanted inflammatory and immune responses due to “naked” RNA exposure, emphasizing the importance of physical LNP stability.21 While such leakage is largely avoided with current formulations, the use of PEGylated lipids represents a long-standing safety concern, despite the fact they are universally incorporated into the existing approved LNPs for RNA delivery.
Originally, PEGylated lipids were introduced as a “stealth sheath” to shield the particles from protein binding and prevent aggregation, thereby reducing clearance and extending plasma half-life.4,21 It is now also recognized they make a key contribution to LNP stability; but on the other hand, PEGylation reduces the cellular uptake and transfection efficiency of RNA-loaded LNPs.22,23 The current solution to balance these conflicting properties is to use “sheddable” PEG lipids, with variation of the carbon chain length of the lipid portion such that the PEGylated component gradually dissociates from the LNP at an optimal rate, representing the best trade-off between particle stability and clearance.21,24
Even with a carefully controlled serum half-life, PEGylated lipids can still induce anti-PEG antibodies and provoke an immune response.25 Moreover, antibody binding to PEGylated LNPs can prematurely release part of the mRNA payload, potentially exacerbating clinical hypersensitivity reactions that can range from mild to severe, or even be life-threatening.26-29 For situations requiring repeat administration, anti-PEG antibodies may lead to more rapid clearance of the drug product and significantly reduce its efficacy.30 And although it is rarely addressed in the context of LNPs, PEG polymers are notoriously prone to oxidation, for example, through the action of serum alcohol dehydrogenase.31,32
Unsurprisingly, the aforementioned shortcomings have stimulated an active search for PEG alternatives. In a notable recent study by BioNTech, polysarcosinylated LNPs exhibited lower inflammatory and immune responses than their PEGylated equivalents when used for mRNA delivery.33 Another interesting strategy is polysialylation, which has already been applied in clinical trials of polysialylated therapeutic proteins without evidence of immunogenicity, and did not induce antibodies when used as an alternative to PEGy-lation for liposomes.34,35
It is also important to consider the ionizable lipid component. Early LNP formulations used permanently charged cationic lipids to achieve nucleic acid delivery, but although they resulted in efficient transfection, their significant cytotoxicity and immunogenicity soon became clear, and proinflammatory effects were often observed.5,36,37 These findings inspired development of more biocompatible ionizable lipids that are neutral at normal physiological pH but become positively charged under the more acidic conditions in late endosomes, thereby promoting escape and cytoplasmic delivery of the RNA payload.38
Because they represent a key milestone for clinical success of LNP-mediated RNA delivery, ionizable lipids have remained a major research focus in the field and are now known to also influence cellular uptake.39,40 Nevertheless, some safety concerns remain, as illustrated by the Packer et al. study mentioned earlier, which identified inactivating lipid–RNA adducts in COVID-19 mRNA vaccines (linked to oxidation of the ionizable lipid component).16,41 Another group found highly proinflammatory effects of LNPs used in preclinical COVID-19 vaccine studies, which were specifically attributable to the ionizable lipid component (likely referring to the ALC-0315 lipid used in the final Pfizer/BioNTech product 6). In addition, ionizable lipids appear to modulate immune responses to LNPs in ways that are not yet well understood.37,38,42 The ability of LNPs to act as both delivery vehicles and adjuvants offers important opportunities, but it will be important to find an optimal balance between the positive adjuvant and negative proinflammatory properties as the field of mRNA vaccines moves forward.
At SiSaf, we created our organic-inorganic hybrid sshLNPs as a well-balanced delivery system to help address this need. Due to their silicon-stabilized design, they are less reliant on PEGylated lipids than conventional LNPs, to the extent that some formulations are entirely free of PEGylation. As well as circumventing the potential aforementioned adverse effects, this makes sshLNPs more compatible with lyophilization and avoids the requirement for cold supply chain distribution. Moreover, compared with existing delivery vehicles that contain cationic lipids (such as liposomes), the silicon stabilization of Bio-Courier may enhance transfection efficiency through alternative surface charge functionalization (by using doped silicon), thus reducing the cationic lipid requirements. Finally, the hydrolyzable silicon component of sshLNPs is designed to degrade in vivo to 100% biocompatible orthosilicic acid, which is readily excreted in the urine and has no known safety concerns.43,44
KEY CHALLENGE #3: TISSUE-SPECIFIC TARGETING
It is well established that LNPs have a strong tendency to accumulate in the liver due to interaction with apolipoprotein E and consequent uptake by hepatocytes, mediated primarily via the low-density lipoprotein receptor (LDLR).45,46 Importantly, overcoming this limitation was recently identified as a critical factor for future growth of LNP-enabled nucleic acid therapeutics.47 While current formulations do allow for LNPs to be directed to spleen or lung in addition to the liver, tissue- or organ-specific targeting beyond this remains a considerable challenge and generally requires optimization on a case-by-case basis.48
One reason for this complexity is the formation of a biomolecular corona around LNPs after administration, through binding of endogenous proteins and lipids to the particles on entering the circulation. This can significantly impact the pharmacokinetics, tissue distribution, and targeting characteristics of the formulation in ways that are difficult to predict. For example, Huayamares et al. screened nearly 100 distinct LNPs to achieve targeted mRNA delivery to tumors without significant liver accumulation.49,50
Bio-Courier formulations can readily be customized, for example, through adjusting the freely modifiable PEGylated lipid content (which can be zero). In principle, this means that more effective tissue targeting may be possible than for standard LNPs, and we have already obtained some evidence for this. In a mouse model of autosomal dominant osteopetrosis type 2 (ADO2), which is caused by heterozygous loss-of-function mutations in the CLCN7 gene, Bio-Courier sshLNPs were able to successfully deliver siRNA to femur, to silence the mutant allele and fully restore normal bone density (Figure 4).51
KEY CHALLENGE #4: TRANSFECTION EFFICIENCY
Robust transfection of target tissues requires both cellular uptake and endosomal escape of the nucleic acid payload, and the low efficiency of the latter remains a major bottleneck for LNP-mediated delivery of therapeutic RNA.40,49 In this regard, ionizable lipids have received the most attention due to their previously explained central role, but recent work has also established the cholesterol component as a major factor. By developing a reporter system to visualize endosomal escape, Herrera et al demonstrated that C24 substituted sterols — particularly β-sitosterol — were much more effective at inducing cytoplasmic delivery of mRNA than unmodified cholesterol.40 Other notable work has established the particle size of LNPs as a major determinant of transfection efficiency, highlighting the importance of robust structural integrity for LNPs intended for clinical use.52 These findings are also reflected in the FDA’s critical quality attributes (CQAs) for liposome drug products, which include particle size and size distribution.53
On a different note, two recent studies have revealed a seemingly underappreciated role for clearly defined stereochemistry in LNP constituents. More specifically, LNPs formulated with stereochemically pure 20α-hydroxycholesterol were found to deliver mRNA three times as efficiently in vivo as those containing mixed isomers, as a result of reduced phagocytic sorting.54 Similar results have just been reported for a chiral ionizable lipid, strongly suggesting that stereochemical integrity is a critical functional factor for LNPs, in line with what has long been known for small-molecule drugs.55
Significantly, the improved physical stability of sshLNPs translates into improved RNA delivery both in vitro and in vivo. In hard-to-transfect human primary cells, standard LNPs failed to deliver an mRNA encoding GFP, while Bio-Courier was able to match the performance of a widely used commercial transfection reagent (Figure 5).56 Furthermore, the Bio-Courier formulations led to sustained transfection for at least 5 days, unlike the commercial reagent.
For in vivo delivery, topical ocular administration of an siRNA-loaded Bio-Courier formulation was found to induce robust corneal gene silencing in luciferase reporter mice (Figure 6).57
KEY CHALLENGE #5: MANUFACTURING
For LNP-mediated delivery of RNA therapeutics, the major logistical challenge is the inherent chemical instability of the RNA itself.12 It may be possible to offset this through future advancements, such as further development of the formulation recently described by Meulewaeter et al, but maintaining RNA integrity currently poses a major problem for clinical applications.20 The issue partly stems from the fact that in existing protocols, the nucleic acid payload must be introduced early in the production process during initial formation of LNPs, prior to subsequent purification and fill/finish steps.58,59 In this approach, the LNPs are effectively formed around the nucleic acid cargo because it cannot easily be introduced later. This limitation not only restricts batch sizes in commercial manufacturing due to the chemical lability of RNA, it also affects the quality of the product that ultimately reaches the patient.60
For example, the Pfizer BNT162b2 COVID-19 vaccine is estimated to lose around 30% of its initial RNA integrity during the production process, with further deterioration expected during distribution and storage — even with an ultracold supply chain.61 Therefore, alternative manufacturing technologies are urgently needed if the full potential of RNA therapeutics is to be realized.62
SiSaf’s sshLNPs offer a significant advantage in this regard because the presence of the silicon component means they remain amenable to nucleic acid encapsulation after initial assembly. In contrast to conventional LNPs, Bio-Courier formulations can be manufactured “empty” and loaded with the desired therapeutic RNA later, at the desired point and time of use. This key difference eliminates the requirement for a cold supply chain since lyophilized or liquid sshLNPs can readily be shipped, reconstituted (if lyophilized), and loaded at ambient temperature. In long-term storage tests, non-lyophilized Bio-Courier sshLNPs maintained their original size and zeta potential for at least 6 months at room temperature and 24 months at 4°C, which was much longer than for conventional LNPs (<12 weeks). Thus, RNA encapsulation and fill/finish operations may be separated from initial manufacture by considerable time and distance, readily permitting on-demand preparation of customized RNA-loaded LNPs on any scale, and at any required dosage for customized patient treatment.
This capability largely eliminates the potential safety and efficacy concerns associated with gradual degradation of RNA during manufacture, distribution, and storage of current formulations. It also opens up previously inaccessible use cases; for example, in personalized medicine (such as cancer mRNA vaccines) or for treatment of geographically constrained diseases.63 The decoupling of RNA encapsulation and LNP formation can significantly reduce costs and environmental impact (e.g., energy demand), and can expand global accessibility to locations where cold chain logistics are not viable. Thus, sshLNPs come with some significant differentiators from current supply chains that mean they could revolutionize the field of nucleic acid medicines.
SUMMARY & OUTLOOK
The global RNA therapeutics market is projected to grow from around $5 billion in 2021 to $25 billion by 2030, yet this relies substantially on improved formulations to maximize the potential of these drugs. While the longstanding use of LNPs in the clinic has firmly established their safety and applicability as a delivery mechanism of choice for nucleic acid drugs, it has also highlighted some important outstanding challenges, such as stability (including storage stability), safety, the targeting of tissues beyond the liver, and the need for RNA encapsulation during LNP formation.64 The hybridization of organic lipids with inorganic biodegradable silicon in Bio-Courier formulations offers potential for improved clinical performance, while reducing the rate of adverse reactions and improving patient safety. As sshLNPs do not require RNA to be encapsulated during initial LNP formation, they open up this line of therapeutics globally without compromising on added energy costs for ultracold transport and storage. This enables an easily accessible kit-based approach, allowing growth of the RNA field not only in vaccines but also in personalized medicine.
REFERENCES
- Wang C, Zhang Y, Dong Y. Lipid nanoparticle–mRNA formulations for therapeutic applications. Acc Chem Res. 2021;54(23):4283–4293. https://doi.org/10.1021/acs.accounts.1c00550.
- Curreri A, Sankholkar D, Mitragotri S, Zhao Z. RNA therapeutics in the clinic. Bioeng Transl Med. 2023;8(1):e10374. https://doi.org/10.1002/btm2.10374.
- Hou X, Zaks T, Langer R, Dong Y. Lipid nanoparticles for mRNA delivery. Nat Rev Mater. 2021;6(12):1078–1904. https://doi.org/10.1038/s41578-021-00358-0.
- Kumar R, Chalarca CFS, Bockman MR, et al. Polymeric delivery of therapeutic nucleic acids. Chem Rev. 2021;121(18):11527–11652. https://doi.org/10.1021/acs.chemrev.0c00997.
- Moss KH, Popova P, Hadrup SR, Astakhova K, Taskova M. Lipid nanoparticles for delivery of therapeutic RNA oligonucleotides. Mol Pharm. 2019;16(6):2265–2277. https://doi.org/10.1021/acs.molpharmaceut.8b01290.
- Paunovska K, Loughrey D, Dahlman JE. Drug delivery systems for RNA therapeutics. Nat Rev Genet. 2022;23(5):263–280. https://doi.org/10.1038/s41576-021-00439-4.
- Kulkarni JA, Witzigmann D, Chen S, Cullis PR, van der Meel R. Lipid nanoparticle technology for clinical translation of siRNA therapeutics. Acc Chem Res. 2019;52(9):2435–2444. https://doi.org/10.1021/acs.accounts.9b00368.
- Saffie-Siebert RS, Torabi-Pour N, Ahmed N, inventors; SiSaf Limited, assignee. A delivery system comprising silicon-containing material. WIPO Patent Application WO 2020/193995 A1. March 30, 2020.
- Saffie-Siebert RS, Baran-Rachwalska PM, Sutera FM, Torabi-Pour N, inventors; SiSaf Limited, assignee. A delivery system comprising silicon nanoparticles. WIPO Patent Application WO 2020/193999 A1. March 30, 2020.
- Saffie-Siebert RS, Ahmed M, Sutera F, inventors; SiSaf Limited, assignee. Compositions comprising doped silicon particles, and related methods. WIPO Patent Application WO 2023/002222 A1. July 22, 2022.
- Saffie-Siebert RS, Welsh M, Torabi-Pour N, inventors; SiSaf Limited, assignee. Nucleic acid vector compositions. WIPO Patent Application WO 2023/002223 A1. July 22, 2022.
12. Schoenmaker L, Witzigmann D, Kulkarni JA, et al. mRNA–lipid nanoparticle COVID-19 vaccines: Structure and stability. Int J Pharm. 2021;601:120586. https://doi.org/10.1016/j.ijpharm.2021.120586. - Zhao P, Hou X, Yan J, et al. Long-term storage of lipid-like nanoparticles for mRNA delivery. Bioact Mater. 2020;5(2):358–363. https://doi.org/10.1016/j.bioactmat.2020.03.001.
- Haas H, Borquez IHE, inventors; BioNTech RNA Pharmaceuticals GmbH, assignee. Stable formulations of lipids and liposomes. US Patent 11,173,120. November 16, 2021.
- Burdette D, Ci L, Shilliday B, et al. Systemic exposure, metabolism, and elimination of [14C]-labeled amino lipid, lipid 5, after a single administration of mRNA encapsulating lipid nanoparticles to Sprague–Dawley rats. Drug Metab Dispos. 2023;51(7):804–812. https://doi.org/10.1124/dmd.122.001194.
- Packer M, Gyawali D, Yerabolu R, Schariter J, White P. A novel mechanism for the loss of mRNA activity in lipid nanoparticle delivery systems. Nat Commun. 2021;12(1):6777. https://doi.org/10.1038/s41467-021-26926-0.
- Blenke EO, Örnskov E, Schöneich C, et al. The storage and in-use stability of mRNA vaccines and therapeutics: Not a cold case. J Pharm Sci. 2023;112(2):386–403. https://doi.org/10.1016/j.xphs.2022.11.001.
- Kamiya M, Matsumoto M, Yamashita K, et al. Stability study of mRNA–lipid nanoparticles exposed to various conditions based on the evaluation between physicochemical properties and their relation with protein expression ability. Pharmaceutics. 2022;14(11):2357. https://doi.org/10.3390/pharmaceutics14112357.
- De A, Ko YT. Why mRNA–ionizable LNPs formulations are so short-lived: Causes and way out. Expert Opin Drug Deliv. 2023;20(2):175–187. https://doi.org/10.1080/17425247.2023.2162876.
- Meulewaeter S, Nuytten G, Cheng MHY, et al. Continuous freeze-drying of messenger RNA lipid nanoparticles enables storage at higher temperatures. J Control Release. 2023;357:149–160. https://doi.org/10.1016/j.jconrel.2023.03.039.
- Kalita T, Dezfouli SA, Pandey LM, Uludag H. siRNA functionalized lipid nanoparticles (LNPs) in management of diseases. Pharmaceutics. 2022;14(11):2520. https://doi.org/10.3390/pharmaceutics14112520.
- Pratsinis A, Fan Y, Portmann M, et al. Impact of non-ionizable lipids and phase mixing methods on structural properties of lipid nanoparticle formulations. Int J Pharm. 2023;637:122874. https://doi.org/10.1016/j.ijpharm.2023.122874.
- Aldosari BN, Alfagih IM, Almurshedi AS. Lipid nanoparticles as delivery systems for RNA-based vaccines. Pharmaceutics. 2021;13(2):206. https://doi.org/10.3390/pharmaceutics13020206.
- Evers MJW, Kulkarni JA, van der Meel R, Cullis PR, Vader P, Schiffelers RM. State-of-the-art design and rapid-mixing production techniques of lipid nanoparticles for nucleic acid delivery. Small Methods. 2018;2(9):1700375. https://doi.org/10.1002/smtd.201700375.
- Shi D, Beasock D, Fessler A, et al. To PEGylate or not to PEGylate: Immunological properties of nanomedicine’s most popular component, polyethylene glycol and its alternatives. Adv Drug Deliv Rev. 2022;180:114079. https://doi.org/10.1016/j.addr.2021.114079.
- Senti ME, de Jongh CA, Dijkxhoorn K, et al. Anti-PEG antibodies compromise the integrity of PEGylated lipid-based nanoparticles via complement. J Control Release. 2022;341:475–486. https://doi.org/10.1016/j.jconrel.2021.11.042.
- Chen W-A, Chang D-Y, Chen B-M, Lin Y-C, Barenholz Y, Roffler SR. Antibodies against poly(ethylene glycol) activate innate immune cells and induce hypersensitivity reactions to PEGylated nanomedicines. ACS Nano. 2023;17(6):5757–5772. https://doi.org/10.1021/acsnano.2c12193.
- Ibrahim M, Ramadan E, Elsadek NE, et al. Polyethylene glycol (PEG): The nature, immunogenicity, and role in the hypersensitivity of PEGylated products. J Control Release. 2022;351:215–230. https://doi.org/10.1016/j.jconrel.2022.09.031.
- Kozma GT, Mészáros T, Vashegyi I, et al. Pseudo-anaphylaxis to polyethylene glycol (PEG)-coated liposomes: Roles of anti-PEG IgM and complement activation in a porcine model of human infusion reactions. ACS Nano. 2019;13(8):9315–9324. https://doi.org/10.1021/acsnano.9b03942.
- Chen B-M, Cheng T-L, Roffler SR. Polyethylene glycol immunogenicity: Theoretical, clinical, and practical aspects of anti-polyethylene glycol antibodies. ACS Nano. 2021;15(9):14022–14048. https://doi.org/10.1021/acsnano.1c05922.
- Musakhanian J, Rodier J-D, Dave M. Oxidative stability in lipid formulations: A review of the mechanisms, drivers, and inhibitors of oxidation. AAPS PharmSciTech. 2022;23(5):151. https://doi.org/10.1208/s12249-022-02282-0.
- Herold DA, Keil K, Bruns DE. Oxidation of polyethylene glycols by alcohol dehydrogenase. Biochem Pharmacol. 1989;38(1):73–76. https://doi.org/10.1016/0006-2952(89)90151-2.
- Nogueira SS, Schlegel A, Maxeiner K, et al. Polysarcosine-functionalized lipid nanoparticles for therapeutic mRNA delivery. ACS Appl Nano Mater. 2020;3(11):10634–10645. https://doi.org/10.1021/acsanm.0c01834.
- Xenetic Biosciences receives program update from partner Shire’s phase 1/2 study evaluating SHP656 in development as a long-acting treatment for hemophilia A. Xenetic Biosciences. Published May 22, 2017. Accessed May 14, 2023. https://www.xeneticbio.com/news-media/press-releases/detail/61/xenetic-biosciences-receives-program-update-from-partner.
- Han X, Zhang T, Liu M, Song Y, Liu X, Deng Y. Polysialic acid modified liposomes for improving pharmacokinetics and overcoming accelerated blood clearance phenomenon. Coatings. 2020;10(9):834. https://doi.org/10.3390/coatings10090834.
- Rietwyk S, Peer D. Next-generation lipids in RNA interference therapeutics. ACS Nano. 2017;11(8):7572–7586. https://doi.org/10.1021/acsnano.7b04734.
- Ndeupen S, Qin Z, Jacobsen S, Bouteau A, Estanbouli H, Igyártó BZ. The mRNA–LNP platform’s lipid nanoparticle component used in preclinical vaccine studies is highly inflammatory. iScience. 2021;24(12):103479. https://doi.org/10.1016/j.isci.2021.103479.
- Swetha K, Kotla NG, Tunki L, et al. Recent advances in the lipid nanoparticle-mediated delivery of mRNA vaccines. Vaccines. 2023;11(3):658. https://doi.org/10.3390/vaccines11030658.
- Bost JP, Barriga H, Holme MN, et al. Delivery of oligonucleotide therapeutics: Chemical modifications, lipid nanoparticles, and extracellular vesicles. ACS Nano. 2021;15(9):13993–14021. https://doi.org/10.1021/acsnano.1c05099.
- Herrera M, Kim J, Eygeris Y, Jozic A, Sahay G. Illuminating endosomal escape of polymorphic lipid nanoparticles that boost mRNA delivery. Biomater Sci. 2021;9(12):4289–4300. https://doi.org/10.1039/d0bm01947j.
- Han X, Zhang H, Butowska K, et al. An ionizable lipid toolbox for mRNA delivery. Nat Commun. 2021;12(1):7233. https://doi.org/10.1038/s41467-021-27493-0.
- Verbeke R, Hogan MJ, Loré K, Pardi N. Innate immune mechanisms of mRNA vaccines. Immunity. 2022;55(11):1993–2005. https://doi.org/10.1016/j.immuni.2022.10.014.
- Kumeria T, McInnes SJP, Maher S, Santos A. Porous silicon for drug delivery applications and theranostics: Recent advances, critical review and perspectives. Expert Opin Drug Deliv. 2017;14(12):1407–1422. https://doi.org/10.1080/17425247.2017.1317245.
- Jurkić LM, Cepanec I, Pavelić SK, Pavelić K. Biological and therapeutic effects of ortho-silicic acid and some ortho-silicic acid-releasing compounds: New perspectives for therapy. Nutr Metab (Lond). 2013;10(1):2. https://doi.org/10.1186/1743-7075-10-2.
- Da Silva Sanchez AJ, Dobrowolski C, Cristian A, et al. Universal barcoding predicts in vivo ApoE-independent lipid nanoparticle delivery. Nano Lett. 2022;22(12):4822–4830. https://doi.org/10.1021/acs.nanolett.2c01133.
- Glassman PM, Myerson JW, Ferguson LT, et al. Targeting drug delivery in the vascular system: Focus on endothelium. Adv Drug Deliv Rev. 2020;157:96–117. https://doi.org/10.1016/j.addr.2020.06.013.
- Verma M, Ozer I, Xie W, Gallagher R, Teixeira A, Choy M. The landscape for lipid-nanoparticle-based genomic medicines. Nat Rev Drug Discov. 2023;22(5):349–350. https://doi.org/10.1038/d41573-023-00002-2.
- Melamed JR, Yerneni SS, Arral ML, et al. Ionizable lipid nanoparticles deliver mRNA to pancreatic β cells via macrophage-mediated gene transfer. Sci Adv. 2023;9(4):eade1444. https://doi.org/10.1126/sciadv.ade1444.
- Niculescu A-G, Bîrcă AC, Grumezescu AM. New applications of lipid and polymer-based nanoparticles for nucleic acids delivery. Pharmaceutics. 2021;13(12):2053. https://doi.org/10.3390/pharmaceutics13122053.
- Huayamares SG, Lokugamage MP, Rab R, et al. High-throughput screens identify a lipid nanoparticle that preferentially delivers mRNA to human tumors in vivo. J Control Release. 2023;357:394–403. https://doi.org/10.1016/j.jconrel.2023.04.005.
- Maurizi A, Patrizii P, Teti A, et al. Novel hybrid silicon–lipid nanoparticles deliver a siRNA to cure autosomal dominant osteopetrosis in mice. Implications for gene therapy in humans. Mol Ther Nucleic Acids. 2023;33:925–937.
52. Chen S, Tam YYC, Lin PJC, Sung MMH, Tam YK, Cullis PR. Influence of particle size on the in vivo potency of lipid nanoparticle formulations of siRNA. J Control Release. 2016;235:236–244. https://doi.org/10.1016/j.jconrel.2016.05.059.
53. Food & Drug Administration. Liposome Drug Products: Chemistry, Manufacturing, and Controls; Human Pharmacokinetics and Bioavailability; and Labeling Documentation; 2018. Docket No. FDA-2016-D-2817. https://www.fda.gov/regulatory-information/ search-fda-guidance-documents/liposome-drug-products-chemistry-manufacturing-and-controls-human-pharmacokinetics-and. - Hatit MZC, Dobrowolski CN, Lokugamage MP, et al. Nanoparticle stereochemistry-dependent endocytic processing improves in vivo mRNA delivery. Nat Chem. 2023;15(4):508–515. https://doi.org/10.1038/s41557-023-01138-9.
- Da Silva Sanchez AJ, Zhao K, Huayamares SG, et al. Substituting racemic ionizable lipids with stereopure ionizable lipids can increase mRNA delivery. J Control Release. 2023;353:270–277. https://doi.org/10.1016/j.jconrel.2022.11.037.
- Chong ZX, Yeap SK, Ho WY. Transfection types, methods and strategies: A technical review. PeerJ. 2021;9:e11165. https://doi.org/10.7717/peerj.11165.
- Baran-Rachwalska P, Torabi-Pour N, Sutera FM, et al. Topical siRNA delivery to the cornea and anterior eye by hybrid silicon–lipid nanoparticles. J Control Release. 2020;326:192–202. https://doi.org/10.1016/j.jconrel.2020.07.004.
- Nag K, Sarker MEQ, Kumar S, et al. DoE-derived continuous and robust process for manufacturing of pharmaceutical-grade wide-range LNPs for RNA-vaccine/drug delivery. Sci Rep. 2022;12(1):9394. https://doi.org/10.1038/s41598-022-12100-z.
- Cameau E, Zhang P, Ip S, Mathiasson L, Stenklo K. Process & analytical insights for GMP manufacturing of mRNA lipid nanoparticles. Cell Gene Ther Insights. 2022;8(4):621–635. https://insights.bio/cell-and-gene-therapy-insights/journal/article/2515/Process-analytical-insights-for-GMP-manufacturing-of-mRNA-lipid-nanoparticles.
- Catignol P, Lim H. Overcoming bottlenecks in RNA manufacturing. Pharma’s Almanac. Published October 24, 2022. Accessed July 2, 2023. https://www.pharmasalmanac.com/articles/overcoming-bottlenecks-in-mrna-manufacturing.
- Daniel S, Kis Z, Kontoravdi C, Shah N. Quality by design for enabling RNA platform production processes. Trends Biotechnol. 2022; 40(10):1213–1228. https://doi.org/10.1016/j.tibtech.2022.03.012.
- De A, Ko YT. A tale of nucleic acid–ionizable lipid nanoparticles: Design and manufacturing technology and advancement. Expert Opin Drug Deliv. 2023;20(1):75–91. https://doi.org/10.1080/17425247.2023.2153832.
- Sahin U, Türeci Ö. Personalized vaccines for cancer immunotherapy. Science. 2018;359(6382):1355–1360. https://doi.org/10.1126/science.aar7112.
- RNA based therapeutic market update 2023: Projected to cross market value of USD 25.12 billion by 2030. PharmiWeb.com. Updated May 24, 2023. Accessed June 15, 2023. https://www.pharmiweb.com/press-release/2023-05-24/rna-based-therapeutic-market-update-2023-projected-to-cross-market-value-of-usd-2512-billion-by-20.
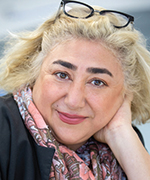
Dr. Suzanne Saffie-Siebert is Founder & CEO, SiSaf Ltd, and the inventor of the company’s proprietary Bio-Courier technology platform. She has over 25 years of diversified pharmaceutical industry experience and is one of the pioneers of using drug delivery carriers for nucleic acids. Her previous leadership positions include Director of Research at pSiMedica Ltd (spin out from QinetiQ) and Head of the Drug Delivery Centre at Dompé SpA (Italy). Suzanne earned her PhD from the School of Pharmacy at the University of London and is inventor or co-inventor of numerous drug delivery patents. She also obtained a Business in Bioscience Diploma from Oxford Brookes University Business School.
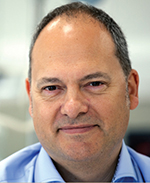
Dr. Michael Welsh is Chief Scientific Officer, SiSaf Ltd. He is an expert in disease stage and biological models and trained as a virologist and immunologist in human and animal health. Since joining SiSaf in 2014, he has worked on a broad range of pre-clinical and clinical programmes involving Bio-Courier technology. He was previously Head of the Virology Department in a UK Government research institute and obtained his qualifications (first class honours degree and PhD in microbiology) from Queen’s University Belfast.
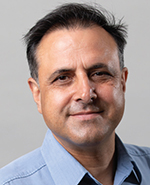
Dr. Nissim Torabi-Pour is Chief Technical Officer, SiSaf Ltd and has played an important role in the development of Bio-Courier technology since the early research phase. Prior to joining SiSaf, he worked as Technical Project Manager and as Project Director at various Biotech companies including pSiMedica Ltd and Global Technologies (NZ) Ltd. His academic research included work on cancer and inflammatory diseases at the Mayo Clinic, USA, and the Royal London Hospital, and on solid and semi-solid drug delivery formulations at Jena University in Germany. Dr. Torabi-Pour earned his PhD at the School of Medicine and Dentistry, University of London.
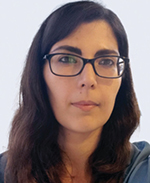
Dr. Flavia M. Sutera is Head of Preclinical Development, SiSaf Ltd, responsible for the preclinical development of SiSaf’s gene therapy pipeline. She has been a key member of SiSaf’s R&D team since 2016, leading many of the laboratory activities related to Bio-Courier design and optimization, both in-house and with academic partners. Dr Sutera obtained her PhD in Experimental Medicine and Neuroscience from the University of Palermo, Italy.
Total Page Views: 3781