Issue:June 2022
PLATFORM TECHNOLOGY - Confident Silence: Delivering on the Promise of siRNA Therapies
INTRODUCTION
Thirty years ago, I moved from medical practice in rheumatology to industry because I wanted to help develop targeted medicines that would treat patients more effectively and safely than the available options. The same aim, amplified by the potential of targeted therapies based on gene silencing (antisense), motivated my move to Silence Therapeutics, which is developing siRNA therapies for many diseases.
The great promise of siRNA is its ability to hit “undruggable” targets – those not amenable to small molecules or biologics. siRNA are targeted medicines that are also precision medicines, because they engage and silence their mRNA targets via the precise mechanism of Watson-Crick base pairing.
Additionally, conjugation of siRNAs to the amino-sugar molecule GalNAc enables highly specific delivery of siRNAs to the liver, which expresses thousands of genes. This exquisite targeting mechanism, elucidated within the past decade, coupled with the precision of siRNA molecules themselves, has opened the door to a whole new arena of targeted therapies, with the first siRNA product receiving regulatory approval in 2018 and two others following suit since then.
As a physician, I find siRNA technology exciting for its potential to create therapies for many diseases, especially rare diseases, which lack effective treatments. As a drug developer, I find it appealing because it provides me with added confidence, well before a product enters clinical testing, which is uncommon for other therapeutic modalities.
THE CAUSES FOR CONFIDENCE
The selection of diseases and gene targets for siRNA therapies can appear daunting because of the myriad possibilities: of the approximately 30,000 genes in the human genome, the liver expresses about 14,000, and only 1% of those are targeted by publicly disclosed siRNAs. This leaves a vast “white space” of potential targets and therapeutic opportunities. When stepping into this space, Silence applies two key criteria to select diseases and targets for our mRNAi GOLD™ platform.
The first criterion is unmet need, which is key to me as a physician. I want to pursue diseases in which patients lack suitable options and develop precision medicines that offer those patients hope and a better, longer life.
The second criterion is a clear “line of sight” from the proposed genetic target to the disease involving that gene. This clarity is possible with siRNA technology because it targets a key enabler of the gene – its mRNA – leading to downstream silencing of encoded protein. By selecting a gene critical to the disease process, we can therefore have confidence that silencing the gene’s mRNA will affect disease progression.
Target selection today is aided by human genetic databases, such as the UK Biobank, that allow us to link a gene to its biological processes and characteristics in humans (phenotypes). We mine these databases to understand whether interfering with a certain gene could treat a disease; and, if so, if other systems might be affected as unwanted side effects. Our goal is to target a gene that has little or no effect on phenotypes outside the disease. In fact, we have made this whole approach to de-risking the target central to our discovery machine.
Lipoprotein(a) or Lp(a), the target of our SLN360 product candidate, is a good example of this. The only phenotype linked to silencing of the LPA gene that encodes the Lp(a) protein relates to the relative risk of cardiovascular disease: high levels of Lp(a) are associated with high risk; low levels are associated with low risk. Some individuals have zero levels of Lp(a) and for them, the only known phenotype is one with a much reduced incidence of cardiovascular events.
After selecting a target gene, we apply machine learning (artificial intelligence) and other in silico techniques to maximize the siRNA’s silencing effect on the target’s mRNA, while minimizing the siRNA’s potential to bind other mRNAs and produce unwanted side or off-target effects. This ability to predict and screen out siRNA sequences that could cause side effects is possible due to the precise mechanism of nucleotide base pairing, and contributes to the wide safety margins we anticipate with our siRNA molecules.
Indeed, while safety is important in treating any disease, minimizing the potential for side effects is especially important in treating chronic diseases, such as hyperlipidemia, where it can take decades for patients to experience any overt symptoms from the condition. Such patients are unlikely to tolerate a therapy with even minor side effects that interfere with their quality of life.
Additional chemistry on our platform links GalNAc ligands to the siRNA to optimize its uptake by liver cells – further adding to safety by reducing or eliminating uptake by tissues not containing the targeted mRNA. This is another feature that sets siRNA therapies apart from small molecule drugs, which often act by targeting proteins and can be highly unpredictable in terms of what tissues they will enter, how they will be metabolized, and which side effects they could have.
Collectively, our developmental approach provides us with a confidence in the safety and efficacy of an siRNA therapy that isn’t always possible with small molecule drugs. That confidence translates to lower clinical failure rates for siRNA therapies: current information tells us that about 50% of siRNAs that successfully complete Phase 1 will successfully complete Phase 3, compared with just 9% for small molecules.
ONE GENE, ONE FUNDAMENTAL PROCESS, MANY RARE DISEASES
In addition to enabling the development of an siRNA therapy for one specific disease, such as hyperlipidemia, understanding the relationship between a gene and its phenotypes can also reveal the possibility of developing a single siRNA product for multiple diseases, especially rare ones, in which the same fundamental biological mechanism is dysregulated.
Iron flux is a good example of a fundamental mechanism that is disrupted in a number of rare diseases. While we depend on iron, in the form of the oxygen-carrying protein hemoglobin in red blood cells, for air exchange, iron itself can be toxic. For this reason, the body has iron-regulating mechanisms that help protect our tissues against toxic iron build-up.
Hepcidin is a key regulator of iron: high levels of hepcidin restrict its availability, while low levels of hepcidin can allow too much of it to circulate. Hepcidin itself is negatively regulated by TMPRSS6, which is the gene target of SLN124: silencing this gene’s corresponding mRNA increases hepcidin expression levels, resulting in lower iron levels into the blood.
The phenotype of TMPRSS6 makes it an attractive target for siRNA. Inherited mutations in the TMPRSS6 gene cause iron-refractory iron deficiency anemia (IRIDA), a type of anemia that does not respond to oral iron therapies; but that is the only phenotype associated with loss-of-function defects in TMPRSS6. This means that silencing TMPRSS6 is not likely to have effects outside of the processes that produce hemoglobin and red blood cells. This validation in terms of human genetics increases confidence in the target both in terms of effect and safety.
In diseases like beta thalassemia and myelodysplastic syndromes (MDS), the body has disrupted the mechanisms that protect it from iron overload. Genetic mutations interfere with the bone marrow’s ability to make normal red cells (erythropoiesis), leading to anemia. Although this anemia is not caused by a lack of iron, the body tries to compensate by releasing additional iron from body stores to help make more red cells. It does this by suppressing the level of the key iron regulator hepcidin. This in turn causes an excess of free iron that poisons the bone marrow and further reduces erythropoiesis, exacerbating the anemia as well as causing problems in other organs, such as the liver, endocrine system, and heart.
The dysregulation of erythropoiesis in beta thalassemia and MDS can also cause splenomegaly (enlarged spleen) for two reasons: the spleen begins to produce red blood cells to compensate for the bone marrow dysfunction; and the spleen has to work harder to remove immature, improperly formed red blood cells from circulation.
We have shown in preclinical animal models of disease that silencing TMPRSS6 to up-regulate hepcidin improves erythropoiesis in the bone marrow, reduces iron overload and spleen size, and increases levels of hemoglobin. Targeting the iron-regulating mechanism to increase hepcidin levels and thereby decreasing iron levels, as Silence’s SLN124 does, could improve erythropoiesis, sparing patients the need for multiple blood transfusions and the risk of organ damage from resulting iron overload. By contrast, iron-chelating therapies do not have all of these therapeutic benefits, because they only “skim” excess iron from the blood without modulating the underlying mechanism that results in that excess.
Polycythemia vera (PV) – our most recently announced program for SLN124 – is another disease where regulation of iron levels by hepcidin can be a therapeutic alternative. PV involves the over-production of red blood cells as a form of cancer, increasing not only red cell mass – the total amount of the cells in the body – but also the overall thickness and stickiness of the blood. This leads to a variety of adverse symptoms, as well as a fourfold higher risk of cardiovascular events, such as heart attacks and strokes.
Increasing hepcidin levels in PV restricts the amount of available iron, effectively placing the bone marrow on a “low-iron diet” that reduces red cell mass, hemoglobin levels, and hematocrit. In a murine transplant model of PV, we and our Australian collaborators have shown that silencing TMPRSS6 reduces these blood markers and the symptoms of the disease.
There are two additional rare diseases, and a widely used treatment procedure, in which increasing hepcidin by silencing TMPRSS6 could be therapeutically beneficial.
One is hereditary hemochromatosis, in which mutations in one of several genes cause excess iron absorption and iron overload. As in beta thalassemia and MDS, increasing hepcidin can treat the disease by reducing levels of iron and its availability.
Another is sickle cell disease (SCD). Studies in preclinical models of SCD have shown that decreasing iron levels reduces the amount of sickling (deformity) of red blood cells and the incidence of sickling-related thrombotic events.
Finally, iron regulation could be beneficial in hematopoietic stem cell transplantation, which is used to treat a range of blood cancers and non-cancerous conditions. This procedure involves ablating the existing bone marrow to make way for the stem cell graft; this ablation shifts a huge load of dead, iron-laden blood cells into the circulation. Retrospective studies suggest the acute release of toxic iron from the ablated cells can adversely affect the survival of the stem cell graft and increase the risk of potentially lethal infections in patients. If levels of the iron could be reduced by up-regulating hepcidin, we believe we could improve survival and engraftment outcomes in hematopoietic stem cell transplant patients.
What I find fascinating and exciting here is that one siRNA therapy has the potential to treat at least five rare diseases and improve outcomes in transplantation procedures to treat many other diseases – all by precisely targeting a single gene regulating a single fundamental process in the liver.
THE NEXT FRONTIERS IN SIRNA
While the liver offers plenty of fertile ground for developing siRNAs therapies to treat many diseases, many disease-related genes are not highly expressed in the liver. For this reason, the next great challenge in the field is delivering siRNA to tissues outside the liver. Finding another targeting ligand with the exquisite specificity of GalNAc for a tissue outside the liver is the “Holy Grail” and likely to be challenging. Meanwhile, researchers and companies are experimenting with various delivery methods: conjugating siRNAs to antibodies or peptide ligands, or loading siRNA into lipid nanoparticles or exosomes.
For many years, I have been passionate about RNA technology in general and, more recently, the benefits that targeted, precision siRNA medicines can bring to patients in need. It is rewarding to see that this technology is finally coming into its own, with the promise of delivering even greater benefits in the near future.
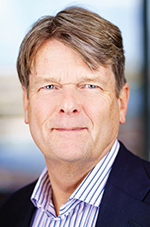
Dr. Giles Campion joined Silence Therapeutics as Head of R&D and Chief Medical Officer in June 2019 and was appointed as an Executive Director in May 2020. He is an expert in translational medicine and a highly experienced biotech and pharmaceutical professional across many therapeutic areas, most recently in orphan neuromuscular disorders. He has held senior global research and development roles in several large pharmaceutical, diagnostics, and biotech companies, including responsibilities at the board level. Dr. Campion previously served as group Vice President, Neuromuscular Franchise at BioMarin Pharmaceutical Inc., or BioMarin, from February 2015 to March 2016, following BioMarin’s acquisition of Prosensa Holding N.V., or Prosensa. He served as Chief Medical Officer and Senior Vice President of Research and Development at Prosensa from 2009 until its acquisition by BioMarin and held executive Research and Development positions at SmithKline Beecham, Novartis and GE-HealthCare. Dr. Campion is also a co-founder of PepGen Ltd. He earned his bachelor’s and doctorate degrees in Medicine from the University of Bristol and is listed on the General Medical Council (UK) Specialist Register (Rheumatology).
Total Page Views: 6087